- Academic Editor
Breast cancer has a special tumor microenvironment compared to other solid tumors, which is usually surrounded by a large number of adipocytes that can produce and secrete fatty acids and adipokines. Adipocytes have a remodeling effect on breast cancer lipid metabolism, while fatty acids and lipid droplets can make breast cancer cells more aggressive. Lipid metabolism, especially the synthesis of fatty acids, is an important cellular process for membrane biosynthesis, energy storage, and signal molecule production. Therefore, blocking the lipid supply to cancer cells or changing the lipid composition has an important impact on the signal transmission and cell proliferation of cancer cells. Alterations in lipid availability can also affect cancer cell migration, induction of angiogenesis, metabolic symbiosis, evasion of immune surveillance, and cancer drug resistance. Fatty acid synthesis and metabolism have received extensive attention as potential targets for cancer therapy, and studies on modulating the tumor lipid microenvironment to improve the sensitivity of antitumor drugs have also been discussed; however, strategies to target these processes have not been translated into clinical practice.
According to Globocan 2020, breast cancer has become the number one incidence of malignancy and now has the fifth highest mortality rate in the world [1]. Breast cancer has a special tumor microenvironment compared to other solid tumors, usually surrounded by a large number of adipocytes that can produce and secrete fatty acids (FAs) and adipokines. Adipocytes have a remodeling effect on breast cancer lipid metabolism, while fatty acids and lipid droplets can make breast cancer cells more aggressive [2].
Fatty acids are a type of lipid with rich and diverse biological functions, acting as both energy storage substances and signaling molecules, as well as key components of the cell membrane structure [3]. An excess of lipids can lead to the onset of metabolism-related diseases, such as obesity, and also promote tumor development, treatment response, and metastasis [4]. Metastasis of tumor cells is a complex process that can be broadly divided into different stages, while cancer cells need to regulate their lipid-related metabolic pathways and trophic structures to adapt to metastasis during different stages. These adaptations comprise altering the composition of lipid membranes to adapt to other microenvironments, altering lipid-associated signaling substances to overcome the mechanisms of cell death, and facilitating lipid catabolism and anabolism to meet energy requirements and oxidative stress protection [5, 6, 7]. Cancer cells can also use lipid metabolism to regulate stromal cell status and immune cell activity, establish tumor cells and the microenvironment [8], develop therapeutic resistance, and promote cancer recurrence.
Thus, lipid metabolism, especially fatty acid metabolism, is an important cell proliferation process. Altered lipid metabolism is an important phenotype of metabolism in cancer cells. Therefore, blocking lipid supply in cancer cells has significant effects on the signal transmission and cell proliferation of cancer cells. In addition, alterations in lipid availability can also affect the tumor microenvironment, thereby influencing cancer cell migration, neovascularization, evasion of immune surveillance, and induction of anticancer drug resistance. Drugs targeting the metabolism of FAs have emerged as a potential therapy for cancer treatment, although therapeutic strategies targeting these metabolic abnormalities have not yet been translated into clinical practice.
Due to the large presence of adipocytes in breast tissue, breast cancer is closely related to the biological function of fatty acid metabolism. Fatty acid metabolism includes multiple pathways, such as fatty acid transport, storage in lipid droplets as triglycerides and cholesteryl esters, mobilization from phospholipids and triglycerides, and fatty acid oxidation. In most human tissues, cellular requirements for fatty acids are generally met through dietary intake, whereas the synthesis of fatty acids from de novo is unimportant, except for in the liver and mammary gland. The differences in the importance of fatty acid synthesis methods in normal versus cancerous tissues make it an attractive therapeutic target [9]. Dysregulation of fatty acid metabolism is thought to play an important role in the malignant transformation of many different cancers, including breast cancer. Key metabolic enzymes involved in fatty acid synthesis and oxidation play a critical role in the proliferation, metastasis, and invasion of breast cancer cells.
There are two types of mesenchymal cells most associated with fatty acid metabolism in breast cancer: Cancer-associated adipocytes (CAAs) and cancer-associated fibroblasts (CAFs). Once CAAs are activated by cancer cells, they secrete higher levels of proinflammatory cytokines, which induce triglycerides to release more fatty acids and metabolic reprogramming that can promote cancer progression [10]. CAFs promote the upregulation of fatty acid transport protein 1 (FATP1) in triple-negative breast cancer cells and contribute to increased uptake of FAs by the tumor microenvironment, which has been shown to promote cancer cell proliferation [11]. Lipid metabolites secreted by cancer-associated fibroblasts can also be taken up by tumor cells to increase tumor cell migration, whereas the knockdown of the fatty acid synthase (FASN) in CAFs, or the use of CD36 antibodies can reduce tumor cell migration [12].
Therefore, the exploration of fatty acids and their metabolism, signaling pathways, and effects on tumors and surrounding cells is of great importance in breast cancer.
Non-essential fatty acids constitute the majority of FAs in our body and are
usually classified into saturated FAs and unsaturated FAs based on their
saturation. FA biosynthesis occurs in the cytoplasm of hepatocytes, mammary
cells, adipocytes, etc., and is mainly catalyzed by FASN, which catalyzes the
conversion of acetyl coenzyme A to palmitate. FASN overexpression and
overactivation are often associated with malignant phenotypes and cancer
progression [13]. Further, palmitic acid can be synthesized as stearic acid and
longer FAs by FA elongase, while overexpression of FA elongase is also associated
with cancer progression [14]. Synthetic fatty acids can be catabolized for energy
production via the
Different degrees of unsaturation can confer very different biological properties on FAs. For example, palmitic acid has a greater impact on tumor development, is proinflammatory and lipotoxic, and can lead to endoplasmic reticulum stress and an increased induction of ROS. Cellular adaptation to obesity is governed by palmitic acid and leads to an enhanced tumor formation capacity by HR-negative breast cancer cells, through increased chromatin occupancy of the transcription factor CCAAT/enhancer-binding protein beta (C/EBPB) and key downstream regulators, including CLDN1 and LCN2 [16]. Conversely, oleic acid, which is mostly stored as triacylglycerol in lipid droplets, is less toxic and has an anti-inflammatory ability and a lower binding affinity for the FA transporter protein CD36—a scavenger receptor in cells that initiate metastasis [17]. However, in some studies, oleic acid induces migration through an EGFR and AKT-dependent pathway in breast cancer cells [18]. Due to the complexity of the organism, different mechanisms by the same fatty acid also require further clarification in future studies. In addition, the FA component of lipid membranes makes them mobile: regions containing short or unsaturated lipids are more mobile, whereas regions filled with tightly packed saturated fatty acids are less mobile. The saturated fatty acid regions allow different proteins to co-localize, and thus, serve as transduction hubs for cellular signals. Highly migratory cells tend to show increased fluidity in lipid membranes, which facilitates the epithelial–mesenchymal transition in cancer cells and their separation from the vasculature and beyond [19]. Conversely, cancer cells with higher membrane rigidity are not sensitive to drug treatment, and oxidative stress induced by chemotherapy drugs or iron-based mechanisms cannot cause damage to cancer cells. In conclusion, differences in the nature and content of these fatty acids influence the effect on cancer cells.
Essential fatty acids refer to fatty acids that are indispensable in maintaining
body function but cannot be synthesized by the body; thus, must be obtained
through the diet (Fig. 1), such as
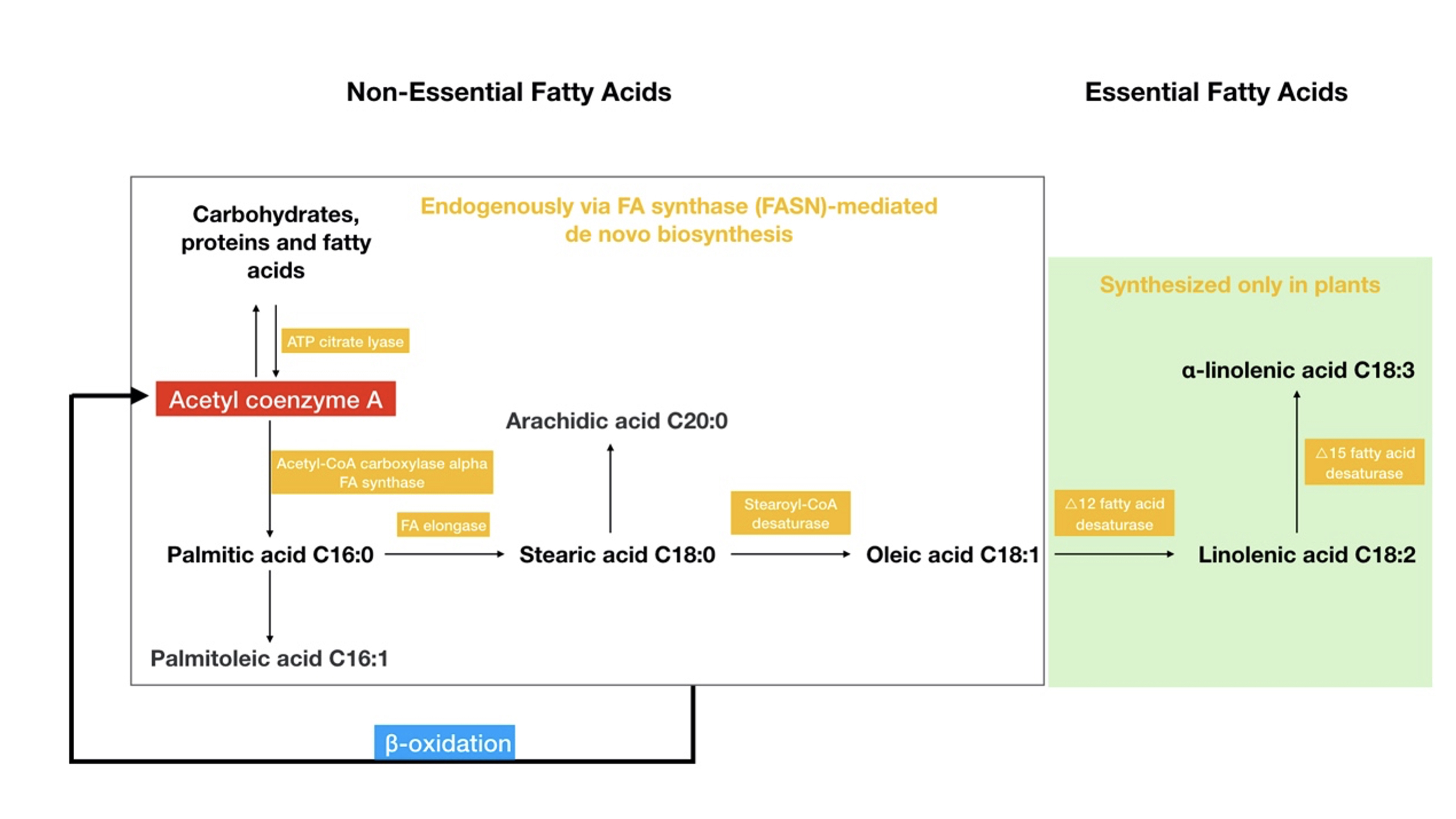
The classification of fatty acids and their metabolism. Non-essential fatty acids can be synthesized in the body, although essential fatty acids can only be synthesized in plants. The figure also shows the relationships between the major fatty acids and the enzymes involved in their metabolism. Acetyl coenzyme A, which acts as a bridge among the three major energy substances (carbohydrates/proteins/fatty acids), is involved in the de novo synthesis pathway of fatty acids, while beta-oxidation of fatty acids also produces acetyl coenzyme A.
Fatty acid type | Main fatty acid | Related pathway or gene | Effect on tumor | Reference | |
Non-essential | |||||
Saturated FAs | Palmitic acid | C/EBPB | Progression | [16] | |
Unsaturated FAs | Oleic acid | EGFR/AKT | Progression | [18] | |
Essential | |||||
Omega-3 fatty acid | SREBP1 | Suppression | [21] | ||
PPAR- |
Suppression | [22] | |||
Omega-6 fatty acid | Linoleic acid | PI3K/AKT | Progression | [20] |
The biological traits and energy metabolism mechanisms in tumor cells are different than in normal cells. It has been found that fatty acids required for the growth and proliferation of malignant tumors are mainly derived from de novo initio synthetic pathways (Fig. 1), and important enzymes related to fatty acid synthesis and oxidative metabolism are overexpressed in tumor tissues, although are not expressed or under-expressed in normal tissues [26]. Overexpression or enhanced activity of ATP citrate lyase (ACLY), an important enzyme in lipid formation related to glucose metabolism, is associated with tumor progression [27]. Decreased ACLY expression limits tumor cell proliferation and survival and inhibits tumor cell invasion and metastasis [28]. The fatty acid rate-limiting enzyme acetyl-CoA carboxylase alpha (ACACA) is highly expressed in breast cancer, and ACACA depletion reduces fatty acid synthesis and induces apoptosis in breast tumor cells, although not in non-malignant cells [29]. Fatty acid synthase (FASN) is another key enzyme in regulating the de novo synthesis of fatty acids. FASN expression is necessary for lipid synthesis and maintenance of fatty acid metabolism levels, especially in the setting of nutritional abnormalities lacking exogenous lipid intake. It has been shown that FASN is required for breast tumor growth and may mediate the occurrence of brain metastases, which can be used as a potential therapeutic target for breast cancer brain metastases [30]. Stearoyl-CoA desaturase (SCD) is a membrane protein of the endoplasmic reticulum (ER) that catalyzes the formation of monounsaturated fatty acids from saturated fatty acids. SCD is overexpressed in cancer cells and controls breast cancer cell proliferation by inducing apoptosis and cell cycle arrest, and preventing migration, although the antitumor effects of SCD inhibitors can be reversed by exogenous oleic acid [31]. SREBP1, an important transcription factor that regulates fatty acid synthesis, is overexpressed in breast cancer and is associated with reduced survival in breast cancer patients [21]. SREBP1 was demonstrated to be significantly associated with epithelial–mesenchymal transition and promotes breast cancer growth and metastasis [32].
Fatty acid
The application of exogenous fatty acid uptake requires crossing cell membranes, and among the proteins involved in the uptake of FAs, CD36 is a major member of metabolic organization [34]. The CD36-mediated uptake of FAs accounts for more than half of the uptake of all FAs in adipose and muscle tissue in mice [35]. In humans CD36-mediated fatty acid uptake is also important, and FA uptake is significantly reduced by CD36 deficiency [36]. CD36 was identified in tumor cell membranes and is involved in the uptake of fatty acids by the surface membrane in a variety of malignant tumor cells; The knockdown of CD36 expression in mouse models completely ceases the aggressive metastatic behavior of tumor cells [37]. CD36 is also expressed at higher levels in tumor-infiltrating regulatory T cells, and inhibition of CD36 blocks the adaptation of regulatory T cells to the tumor microenvironment and enhances antitumor activity [38].
In conclusion, the specific phenotype of aberrant fatty acid metabolism in tumor cells is gradually attracting great attention, and exploring the role of aberrant fatty acid metabolism in the development of breast cancer and strategies to treat malignant tumors by targeting fatty acid metabolic pathways is gaining attention.
The response and resistance of tumor cells to chemotherapeutic agents have been thought to be associated with alterations in the lipid composition of cell membranes. According to preclinical data, one of the characteristics of the resistance by tumor cells to chemotherapy drugs was the decrease in the fluidity of the membrane lipid bilayer. The decrease in fluidity is due to changes in the proportion of saturated fatty acyl chains in the lipid composition of the cell membrane, which plays a leading role, especially in lipid tumor cells. Due to the reduced fluidity, the drug is blocked from entering tumor cells through passive diffusion or endocytosis, and the absorption may be destroyed [39, 40]. Furthermore, it leads to enhanced formation of membrane domains in resistant regions that activate membrane-bound ATP-binding cassettes (ABCs) multidrug efflux transporters, thereby promoting a multidrug resistance phenotype that affects anticancer drugs other than chemotherapy [41, 42]. Interestingly, the regulation of membrane fluidity by supplementing polyunsaturated fatty acids can change the ABC-mediated drug efflux, which indicates that lipid modulators or dietary intervention may be an effective strategy for chemo-sensitization.
Since chemoresistant cancer cells have a relatively low ratio of polyunsaturated and saturated fatty acids in their cells, they are less sensitive to toxic lipid peroxidation in response to many chemotherapeutic drugs induced by oxidative stress, compared to sensitive cancer cells, thus, inhibit cell apoptosis and ferroptosis [40, 43]. Lipid peroxidation is a major feature of ferroptosis, and glutathione peroxidase 4 (GPX4), which is a selenium-containing cysteine enzyme, eliminates lipid peroxidation and prevents cell ferroptosis [44, 45]. A reduction in lipid peroxidation sensitivity appears to be associated with enhanced antioxidant defense, which is characteristic of chemoresistant cancer cells.
As more evidence suggests that membrane changes are associated with chemotherapy drug resistance, key pathways, and enzymes that drive changes in lipid distribution in tumor cells have become potential targets for improving chemotherapy sensitivity. Thus, fatty acid synthases—major members of fatty acid metabolism—can alter the susceptibility of many types of cancer cells to chemotherapy drugs, while ectopic overexpression of FASN in breast cancer cells can generate widespread chemotherapy resistance in vitro [46]. The chemical sensitization of the FA inhibitor orlistat is related to a decrease in the expression of the multidrug resistance protein, thereby indicating that the change in membrane composition may be important. In a phase I clinical trial, oral FASN inhibitor TVB-2640 reverses taxane resistance in breast cancer with an acceptable toxicity and has potential clinical application [47].
Targeting fatty acid oxidation as a chemo-sensitization strategy has also received attention, as it promotes the survival of tumor cells by generating energy and maintaining redox homeostasis. There are significant differences in the expression of CPT1B and CPT1C in recurrent breast cancer and chemotherapy-resistant breast cancer tumor tissues or cell lines compared to primary cancer cells [15, 48]. Indeed, inhibition of fatty acid oxidation using CPT1 inhibitors resulted in sustained chemo-sensitization of tumor cells [15].
Lipid droplet accumulation is another less-studied feature of chemoresistant cancer cell lines [49, 50, 51]. Lipid droplets can serve as an additional source of oxidation of fatty acids under conditions of metabolic stress or as a “sink” for isolating hydrophobic drugs [52], thereby directly contributing to chemoresistance. Likewise, in a model of chemoresistance, breast cancer cell lines were filled with smaller but functional mitochondria and numerous lipid droplets compared to parental cells [51]. Subsequent analysis of these cell lines and clinical chemotherapy-resistant breast cancer cells revealed an enhanced expression of the lipid droplet locus protein recombinant perilipin 4 (PLIN4), a perilipin coating on the observed lipid droplets, which is involved in the mobilization of lipid droplet fatty acids. The transcriptional silencing of PLIN4 reduces the activity of drug-resistant cells but has no effect on drug-sensitive cells, which indicates that targeting PLIN4 may be able to reverse breast cancer chemoresistance.
The use of anti-HER2 targeted therapy can increase the survival of HER2+ breast cancer patients; however, some patients cannot get released from the therapy and some patients develop drug resistance. The intake of essential fatty acids can affect anti-HER2 efficacy and ALA and docosahexaenoic acid, as omega-3 fatty acids, modulate the HER2-associated lipogenic phenotype, induce cell apoptosis, and increase anti-HER2 therapy in HER2-overexpressed breast cancer cells [53]. From the combinational models of the oncoinformatic, pharmacodynamic, and metabolic parameters, long-chain omega-3 fatty acids are potential targeted therapeutics for HER2-positive breast cancer treatment [54]. During the anti-HER2 treatment, modification of nutrient intake during treatment is also a potential strategy to improve the efficacy of drug therapy.
The finding that HER2 signaling pathways activate the expressions of FAs to
promote cancer cell proliferation [55, 56] and that there is bidirectional
crosstalk between these pathways suggests that inhibiting FAs is a reasonable
strategy to conquer the acquired resistance to HER2-targeted therapeutics in
preclinical cancer models [57]. An experiment on tumor-bearing mice showed that
the FASN inhibitor G28UCM alone or in combination with trastuzumab can
significantly improve the anticancer effect of HER2+ breast cancer or
trastuzumab-resistant HER2+ breast cancer without the same side effects caused by
In vitro, the most striking feature of breast cancer cell resistance to lapatinib (HER2 and EGFR inhibitor) is the upregulation of CD36, and in turn, the uptake of fatty acids is accompanied by lipid droplet accumulation. After clinical patients received HER2-targeted therapy, the expression of CD36 in clinical breast cancer tissues was also significantly increased, and the clinical prognosis of HER2-positive breast cancer with higher CD36 levels was poor, indicating that fatty acid uptake and metabolism were related to anti-HER2 targeted therapy resistance [59]. Re-sensitization of lapatinib-resistant cells and the induction of apoptosis by knockdown or pharmacological inhibition of CD36. The role of CD36 in lapatinib resistance was also supported by mouse tumor transplantation models, whereby anti-CD36 antibodies significantly sensitized drug-resistant tumors to lapatinib [60].
Hormones such as estrogens and androgens act in concert with their anabolic effects, profoundly affecting their target tissues as well as lipid metabolism in hormone-dependent breast cancers. The positive efficacy of endocrine therapy in the treatment of locally recurrent or metastatic diseases reflects the dependence of hormone receptor-positive breast cancer cells on these hormone-signaling pathways, although endocrine resistance frequently occurs. Analysis of endocrine-resistant breast cancers has shown prominent features of lipid metabolism upregulation in transcriptomic or proteomic comparisons [61, 62]. Studies of lipid-modifying drugs with endocrine therapies have shown good preclinical efficacy, yet these observations lack clinical support.
In ER-positive breast cancer, the interaction between estrogen signaling and
lipid metabolism is not clear because of the presence of two homologous receptors
(ER
Fatty acid metabolism has potential prognostic significance in cancer and has an impact on the remodeling of the tumor microenvironment and the efficacy of immunotherapy [65]. Regulatory T lymphocytes are essential for human immune tolerance but can also promote immunosuppression and immune escape from the tumor microenvironment, thereby diminishing the efficacy of tumor immunotherapy. Moreover, it has been reported that lipid signaling promotes the functional specialization of tumor-regulating T lymphocytes. The study shows that inhibition of lipid synthesis and metabolic signaling depends on the SREBP of regulatory T lymphocytes to generate an effective antitumor immune response without autoimmune toxicity [66]. This study also found that SREBP activity was significantly higher in tumor-regulating T lymphocytes for human breast or head and neck squamous cell carcinomas. In addition, the deletion of the SREBP cleavage activator protein, which is required for SREBP activity in these cells, inhibited tumor growth and enhanced the immunotherapeutic effect of PD-(L)1 inhibitors targeting programmed death proteins on the surface of killer T lymphocytes. The de novo synthesis of fatty acids from fatty acid synthase contributes to the functional maturation of regulatory T lymphocytes [67], while the absence of fatty acid synthase from regulatory T lymphocytes can inhibit tumor growth.
FASN has recently been found to be associated with increased PD-L1 expression, thereby suggesting a role for fatty acids in the impairment of the immune response in the tumor microenvironment [68]. Orlistat, an agent used in the treatment of obesity, has been reported to reduce FASN activity, as well as tumor growth activity in different cancer models. The drug produced a significant decrease in PD-L1 expression. These findings suggest that orlistat interferes with different mechanisms involved in the control of tumor cell growth and may contribute to the reduction of tumor-associated immunopathogenesis. It was also found that the tumor microenvironment was altered in PD-(L)1-acquired resistant tumor cell lines. Furthermore, Serpinf1, a protein that codes for pigment epithelial-derived factor (PEDF) was further explored. Serpinf1 overexpression was associated with increased production of free fatty acids and reduced activation of CD8+ cells. Conversely, the drug reduced free fatty acid production and reversed resistance to anti-PD-(L)1 therapy [69].
PD-1 signaling alters metabolic reprogramming and contributes to fatty acid
utilization in
Nanocarrier-based drug delivery systems have been widely used in the clinic to improve drug targeting and effective drug concentration. Lipid-based drug delivery has also found excellent application in the treatment of breast cancer. Drugs already in clinical use include liposomal adriamycin and liposomal paclitaxel, which can improve therapeutic efficacy and reduce toxic side effects. By changing the fatty acid composition in nanostructured lipid carriers, structural modifications, such as docosahexaenoic acid-based nanostructured lipid carriers [71], linoleic acid-coupled chitosan nanoparticles [72], and palmitate albumin nanoparticles [73] can influence drug delivery, alter drug sensitivity, reverse therapeutic resistance, and reduce toxic side effects. The combination of nanostructured lipid carriers with raloxifene, a previous endocrine therapeutic drug due to poor oral bioavailability, has enhanced the bioavailability and improved antitumor efficacy.
Owing to the specificity of the tumor microenvironment of adipocyte infiltration around breast cancer, a growing number of studies recognize the impact of fatty acid metabolism on tumor progression. Fatty acid homeostasis can alter cell viability and change lipid membrane composition, thereby affecting membrane fluidity and permeability, leading to tumor cell motility and metastasis. Fatty acid metabolism has also been associated with acquired resistance to a variety of anti-breast cancer drugs. Importantly, numerous studies have recently pointed out that combination therapeutic strategies targeting fatty acid metabolism to overcome therapeutic resistance may be a viable approach for the future, especially in the context of abnormal lipid metabolism and metabolic dysfunction. Finally, we believe that the use of tumor genomic testing in combination with classifying fatty acids in tumor cells, the microenvironment, and daily diets, to design more comprehensive precision medicine strategies, will be the direction to improve the prognoses of breast cancer patients.
XZ and JR designed the research. MH and SX searched the literature and wrote the manuscript. FY provided help and advice on the manuscript structure. All authors contributed to editorial changes in the manuscript. All authors read and approved the final manuscript. All authors have participated sufficiently in the work and agreed to be accountable for all aspects of the work.
Not applicable.
Not applicable.
This research was funded by Educational Commission of Zhejiang Province of China Funder, grant number Y202043475.
The authors declare no conflict of interest.
Publisher’s Note: IMR Press stays neutral with regard to jurisdictional claims in published maps and institutional affiliations.