- Academic Editor
During pregnancy, the Fetal Membrane (FM) is subjected to mechanical stretching that may result in preterm labor. The structural integrity of the FM is maintained by its collagenous layer. Disconnection and reconnection of molecular bonds between collagen fibrils is the fundamental process that governs the irreversible mechanical and supramolecular changes in the FM. At a critical threshold strain, bundling and alignment of collagen fibrils alter the super-molecular structure of the collagenous layer. Recent studies indicate that these changes are associated with inflammation and/or expression of specific proteins that are known to be related to uterine contractions and labor. The potential healing of stretching-induced damages in the FM by mediators involved in mechano-transduction is discussed.
Preterm Birth (PTB) is the leading cause of neonatal morbidity and mortality, accounting for 70% of perinatal mortality and nearly 50% of long-term neurological morbidity [1]. The latter includes neurodevelopmental handicaps, chronic respiratory problems, infections, and ophthalmological problems [2]. Preterm birth puts infants at increased risk of death during infancy and contributes to developmental delays [3].
PTB is described as a syndrome caused by various etiologies associated with multiple mechanisms of disease [4]. Specific maternal tissue and organ systems, such as the cervix, myometrium, and decidua, and fetal organ systems, such as the fetal membranes (amniochorion) and placenta, synchronously contribute to pregnancy wellbeing and ensure protection from all exogenous and endogenous factors [4, 5].
The risk of PTB is particularly high in pregnancies associated with uterine over-distention and particularly in multiple pregnancies. The latter account for 3% of all births but 17% of births before 37 weeks’ gestation and 23% of births before 32 weeks [1]. This information indicates a possible relationship between mechanical stresses applied on different uterine organs/tissues, particularly the fetal membrane (FM) and PTB.
During the gestation period, the fetus is enclosed by the FM. The membrane undergoes several mechanical adjustments to support the load of both the fetus and the amniotic fluid. The mechanical adjustments in the membrane facilitate bearing the local deformation associated with fetal movement and growth [6]. Except for a small region of the FM located above the cervix, the FM is basically encompassed by the thick uterine muscle, which endures most of the mechanical loads [7, 8]. Physiological and sometimes pathological intrauterine pressure induces bi-axial mechanical stretching of the FM, particularly above the uterine cervix region. Therefore, there is particular interest in studying the mechanical behavior of the FM during stretching.
As shown in Fig. 1, the FM is a bilayer structure composed of the amnion and chorion layers.
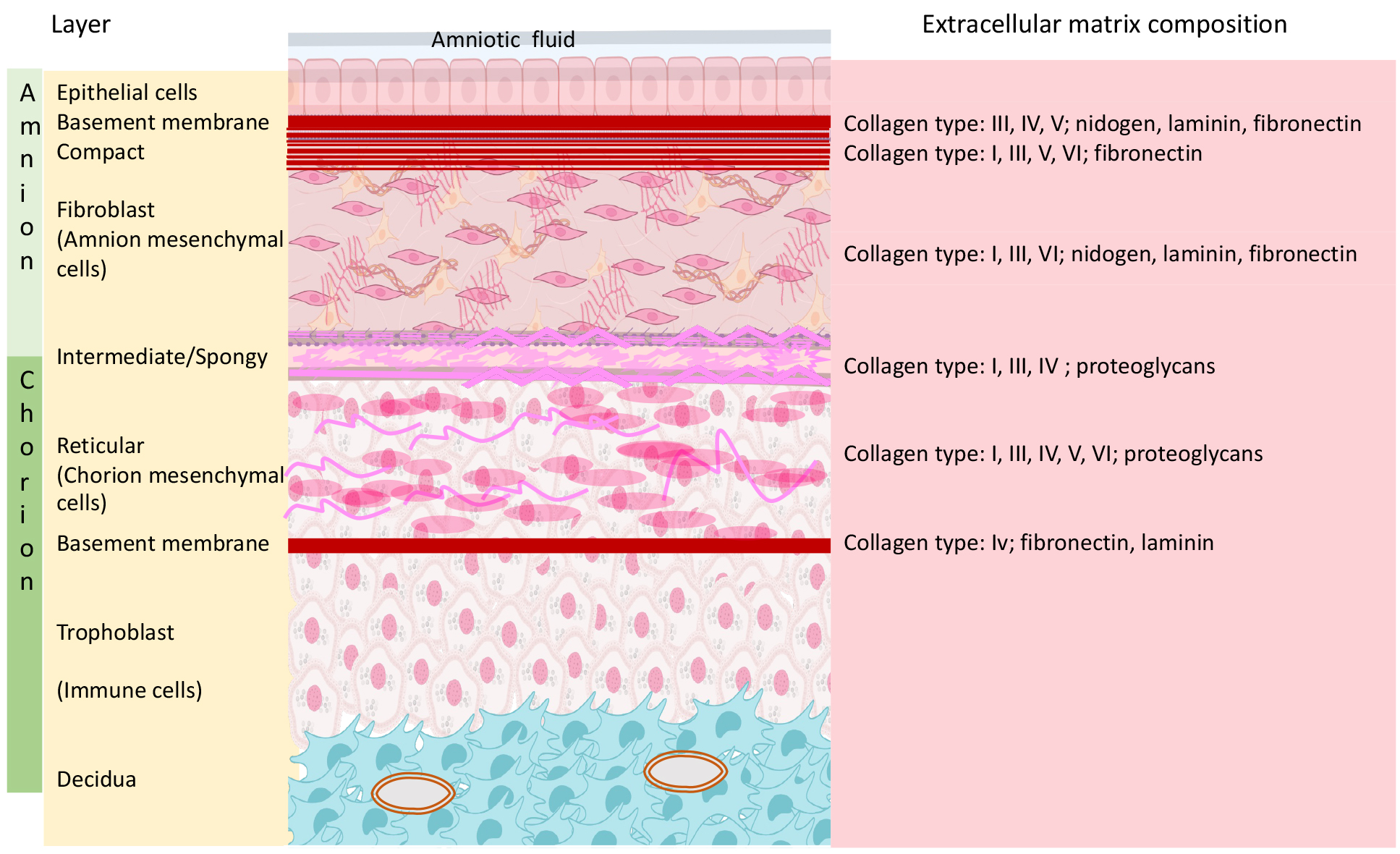
Fetal membrane, cartoon presentation of the layers, and extracellular composition. Schematic illustration of the 2 basic layers (Amnion and Chorion) and the 3rd layer (Choriodecidua) which is composed from the most outer part of the fetal chorion layer and the maternal decidua. Each of the basic layers has different cellular and extra cellular components.
The chorion layer is thicker than the amnion (413 µm versus 111 µm), but the latter is stiffer and stronger and thus dominates the mechanical response of the FM, even though it only accounts for approximately 20% of the FM thickness [6, 9, 10, 11, 12]. The amnion is avascular and comprises five sub-layers, and the nutrients it requires are supplied by the amniotic fluid [11, 12]. The relatively high stiffness and strength of the amnion are attributed to the distribution of collagen in the connective tissue, particularly in the compact layer [13].
The innermost layer, nearest the fetus, is composed of epithelial cells, which secrete collagen types III and IV forming the subsequent basement membrane. The compact layer of connective tissue, composed of collagen, predominantly Types I and III, is adjacent to the basement membrane, forming the fibrous framework of the amnion. The collagens of the compact layer form a cross-linked network with no distinct directionality in the layer plane and are responsible for the mechanical integrity of the amnion [10, 14]. Below the fibroblast layer is the intermediate spongy layer, which lies between the amnion and the chorion layer and allows the amnion to slide on the underlying chorion [10, 13]. The chorion layer is firmly adhered to the decidua, the maternal uterine inner lining, therefore called also the “choriodecidua” layer. When the fetal membranes separate from the uterus at delivery, part of the decidua remains attached to the chorion [14].
The biomechanical mechanisms for the preservation and the disintegration of the FM have been studied for more than 30 years [6, 7, 9, 10, 11, 12, 13, 14, 15, 16, 17, 18, 19, 20, 21, 22, 23, 24]. Oxlund et al. [15] in 1990 used a materials-testing machine and stated that the load-strain relationship of the intact chorioamniotic membrane is that of a two-component material, composed of the less extendable and stronger amniotic component and the more extensible chorionic component.
The mechanical integrity of the chorioamnion membrane, as well as the component chorion and amnion layers, was assessed by Oyen et al. [6] using biaxial puncture testing. The force needed to puncture the choriodecidua was found to be less than that necessary to puncture the amnion. Still, the amnion was more mechanically sensitive and varied in response at different physical locations within the same patient [6]
In other reports, Oyen et al. [16, 17] described the need for smaller puncture force with more advanced gestational age. This observation was valid in both chorion (CH) and amnion (Amn). Furthermore, the chorion does not seem to be significantly affected by the chemical and mechanical changes that occur during gestation and delivery, whereas the amnion was quite sensitive to these changes [16, 17].
In a more recent study, Bircher et al. [18] demonstrate the superior strength and toughness of Amn compared to CH, as quantified from uniaxial tension to failure tests and mode I fracture experiments. However, the authors concluded that conventional fracture mechanics-based calculations could not predict the tear resistance of Amn for defect sizes up to a few millimeters.
During the rupture of the chorion, the force decreases slightly before increasing again prior to failure, indicating that the amnion layer bears most of the stress. It is also logical to assume that the rupture of amniotic membranes due to stretch forces would involve the biochemically triggered weak zone of the membranes [23]. Uniaxial tensile tests and fracture tests were performed on human amniotic membranes to quantify the mechanical conditions which can fracture the membrane with defects. The fracture toughness of amniotic membranes has been measured, and the measurement is consistent across sample geometry. The results show that amniotic membranes are tough and tolerant to defects [23].
In some cases, preterm births start with a premature rupture of the FM due to etiologies such as inflammation or uterine cervical incompetence. More usually, a rupture of the FM occurs due to strong contractions as a part of the labor process. However, in other cases, preterm birth starts with weaker uterine contractions, which only later lead to the rupture of the FM. One explanation for such a reaction chain is that the precontraction mechanical stretch of the FM leads to a change in the molecular and super-molecular structure of the FM. This change alters the molecular connections of the tissue with its surroundings and may lead to changes in the expression and/or activity of specific genes. The balance between the progesterone receptor isoforms and an increased expression of specific Matrix Metalloproteinases (MMPs) have been suggested to be involved in this process [25, 26, 27, 28]. Such a process can explain the mechanism that links an overdistended uterus to preterm PTB.
The aforementioned hypotheses call for exploring irreversible processes and deformations that occur under stress levels. These irreversible deformations alter the molecular and super-molecular structure of the FM, inducing their disintegration. Perrini et al. [24] and Oyen et al. [16] observed, during the first cycle of strain, a large hysteresis loop (representation of the difference between the paths of the loading and unloading curves, which is associated with energy loss mechanisms such as friction), and irreversible deformations. This effect was followed by a much smaller hysteresis in subsequent cycles at the same strain level. Still, no explanation was given for this phenomenon. Part of the studies on the FM tissues was performed on preconditioned specimens, which were subjected to several cycles of loading and unloading before the test began [8, 9, 19, 23]. In these cases, the first cycle, during which most of the irreversible deformation and collagen fiber rearrangement take place, could not be studied. Jabareen et al. [9], who performed a uniaxial tension test on a preconditioned specimen, found that the difference between two consecutive cycles decreases as the number of cycles increases, and no permanent deformations are reported. Obviously, additional studies are needed to elucidate the phenomena.
New challenges in the field of synthetic materials compelled the U.S. government in 2011 to launch the “Materials Genome Initiative” (MGI) project [29]. The idea was to accelerate the pace of discovery and deployment of advanced material systems, using new strategies to, among other challenges, gain a complete understanding of the relations between (a) atomistic-scale properties which emerge from the basic interactions between atoms/molecules, (b) the resulting microstructure and its evolution under external stimuli (e.g., loads), and (c) the macroscopic behavior and function of the material. It has been suggested that knowledge of these relations can lead to the systematic development of new materials (rather than the trial-and-error approach), reducing the time and cost for these materials to enter the market [30, 31, 32]. For live tissues, the problem is much more complex as the aforementioned factors (a, b, and c) are coupled with biomolecular effects and can be altered by biomolecular processes.
Section 2 of this paper (the mechanical integrity of the FM) will review studies of the relations between the aforementioned factors (a–c) in the FM, from large to small scales; specifically, the macroscale irreversible mechanical behavior of the FM, related to the microscopic rearrangement of collagen fibrils, which are governed by the atomistic-scale interaction energies that determine the disconnection and reconnection of bonds between fibrils. Then, section 3 (Biomolecular Processes in Stretched FM) will review recent works on biomolecular processes which occur during the stretching of the FM, with a focus on the expression of different proteins that play a role during labor.
The mechanical integrity of the amnion is mostly provided by the collagens of the compact layer [12]. Collagenous tissues are typically based on collagen fibrils as elementary building blocks, which are assembled in a complex hierarchical way into macroscopic structures. The lowest level is collagen molecules, which are triple helical protein chains. The collagen molecules are assembled in parallel into fibrils, bounded together by a rich extracellular matrix [33]. The compact layer of the amnion is composed of thin collagen fibrils with diameters around 50 nm [12]. The fibrils form a cross-linked network with no distinct directionality in the layer plane [34]. Collagen structures at different scales can bear different amounts of strain before failure. While a macroscopic collagenous tissue, composed of many fibrils, can accommodate strain levels of tens of percent, a single fibril within the tissue can bear only 2.5% at most. Moreover, a single collagen molecule undergoes 1.5% strain at maximum [35, 36].
Microscopic observations of FM tissues show that most of the collagen fibrils are undulated (not fully straight) and that each fibril has a different amount of undulation [20, 37, 38, 39, 40, 41, 42, 43]. These observations, together with the information obtained from mechanical tests of single fibrils, indicate the suitability of the “recruit” model for simulating the mechanical response of the FM. The recruit model is a general approach for calculating the mechanical response of fibrous tissues based on the following scenario [20, 22, 44]: in a group of collagen fibers, each fiber has a different amount of undulation and is thus straightened at a different level of stretch (Fig. 2a). Initially, the collagen fibers of the FM are undulated. Upon stretching, the fiber that has the lowest amount of undulation straightens first, followed by the fiber with the second lowest amount of undulation, and so on. Thus, as the stretching strain increases, the number of straightened fibers increases. It is assumed that only the straightened fibers carry the load, and therefore the stiffness increases as the strain increases. For a collagenous tissue composed of numerous collagen fibers, the effect of individual fibers cannot be resolved, and a smooth stress–strain curve is obtained (Fig. 2b). Under a cyclic load, some amount of hysteresis is usually observed due to viscous effects in the collagen fibers or the matrix [22, 34, 35]. Even though the response of fully straightened elements is linear, the overall response of this mechanism is nonlinear.
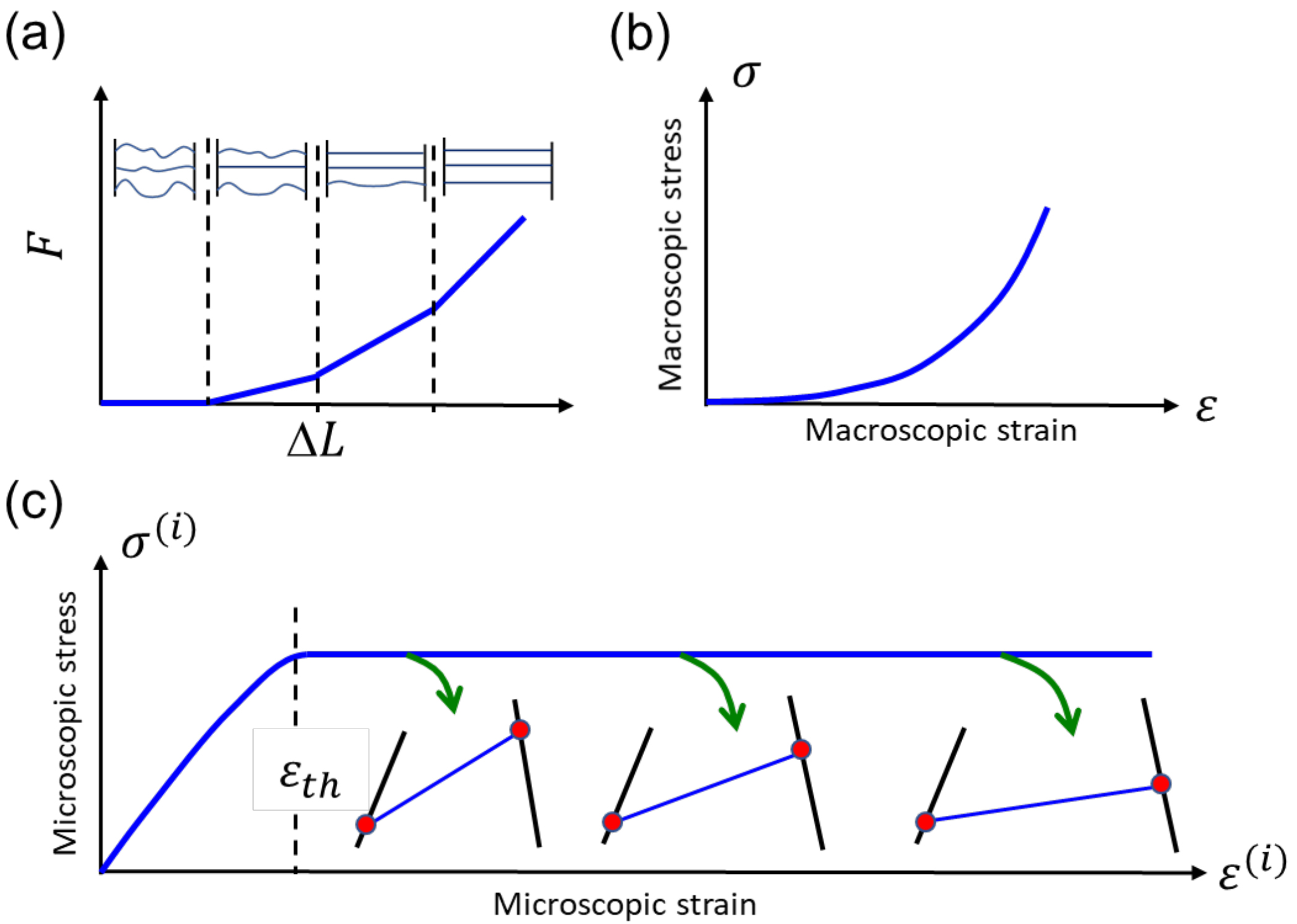
Illustrated stress-strain graphs. (a) Illustration of the basic recruit model, assuming that after straightening, the fibers behave as linear springs. (b) The macroscopic stress–strain curve of a tissue that contains numerous fibers. (c) The stress–strain curve of an individual fibril element. The red dots represent bonds between fibrils (black and blue lines). When these bonds disconnect and reconnect at a different location, the central (blue) fibril is reoriented.
Above a threshold strain
The above-described model has been elaborated considering new findings on correlations between parameters characterizing the mechanical response and parameters quantifying the microstructural constituents. Buerzle et al. [34, 44, 45] described in their studies a relation between the membrane’s strength as well as stiffness at large deformations and the collagen content as well as its cross-linking. Perrini et al. [24] and Mauri et al. [46] described significant volume reduction associated with dehydration of the FM during loading. This effect was mostly recovered during unloading.
The above-mentioned model and experimental results were still not focused on mechanisms for irreversible deformation and recovery occurring during loading and unloading, respectively. In a more recent study from our lab, bulge tests were performed on ex vivo FM tissues, simulating the mechanical conditions prior to contractions. Experimental results revealed an irreversible mechanical behavior that appears during loading and is significantly different than the mechanical behavior that appears during unloading or in subsequent loading cycles. The irreversible behavior results in a residual strain that does not recover upon unloading and remains the same for at least 1 h after the FM is unloaded. Surprisingly, the irreversible behavior demonstrates a linear stress–strain relation. A new model was presented for the mechanical response of collagen tissues, which is based on the recruit model approach, but accounts for the irreversible deformation and provides predictions in agreement with the experimental results [47].
The basic assumption of the model is that the constitutive stress–strain
relationship of individual elements which make up the collagen fibrils has a
plateau segment, during which an irreversible deformation occurs, as illustrated
in Fig. 2c. Fittings of calculated and measured stress–strain curves reveal a
well-defined single-value property of collagenous tissues, which is related to
the threshold strain of individual elements (
Stress-induced collagen rearrangement within the compact layer of ex vivo amniotic membranes was also documented in our lab [48]. The observed collagen bundling and alignment indicate deviations from the expected equibiaxial stress state. The statistical analysis of the fiber orientations clarified the effects of two sources: microscale flaws, which result in local fiber alignment along various and arbitrary directions, and a small uncontrolled macroscale deviation from the equibiaxial strain, which results in global alignment along a specific preferred direction.
Our results imply that at high strains, the macroscale effect becomes dominant. We introduced a model that explains the macroscopic process in terms of a competition between the two effects which occur due to collagen fiber alignment. The first effect is a transformation (plastic) strain, which results in stress relaxation and acts to revert the stress state to the equibiaxial mode. The second effect is a change of the elastic moduli along different directions, such that the modulus along the fiber alignment direction is increased at the expense of the modulus along the perpendicular direction. The resulting elastic modulus anisotropy acts to increase the deviation from the equibiaxial stress state. The model calculations demonstrate how different values of the material properties result in significantly different mechanical behaviors of the collagenous membrane. Moreover, for certain combinations of material properties, a small deviation from the equibiaxial strain conditions results in substantial alignment of collagen molecules along the preferred direction. The results of this study indicate that a temporal increase in the FM stress, which may be induced either by an increase in intrauterine pressure or by an increase in the cervix opening, may lead to irreversible changes in collagen arrangements at both the microscopic and macroscopic scales.
The irreversible mechanical behavior of ex vivo FM at different temperatures and strain rates was further studied in our lab [49]. The analysis assumed that the irreversible deformation is governed by the disconnection and reconnection of bonds between fibrils, as illustrated in Fig. 2c. This process is known to have an important role in the mechanical behavior of collagenous tissues; specifically, in the FM, this process leads to rearrangement and bundling of fibrils [26, 40]. At the same time, the contributions of other sources, such as the behavior of other (softer) layers of the FM and the non-affine deformations between collagen fibrils and Glycose-amino-Glycans (GAG) [50], are yet to be studied. The activation enthalpy of bonds between fibrils was found to be H = 1.24 eV, with a standard deviation of 0.43 eV. This result is close to the value of 1.1 eV reported in a previous study that investigated the activation enthalpy of collagen fibers in bones subjected to uniaxial stress [51]. The obtained activation enthalpy of interfibrillar bonds matches the typical enthalpy values of polyvalent ionic bonds.
Previous studies suggested that the source for negative charges is proteoglycan
proteins, such as decorin or biglycan [51]. The FM, and the amnion specifically,
is very rich in proteoglycan proteins, which consist of several units that are
covalently attached to GAG chains. Two of the most common proteoglycan proteins
in the FM are decorin and biglycan, and both were found to be connected to the
premature rapture of the FM [52, 53]. Moreover, the amnion has a large amount of
decorin, located in close connection with the collagen fibrils [54]. The
extracellular matrix between collagen fibrils is rich in several different
cations, including Ca
Studies on the rupture of term membranes should be carefully appraised when
discussing preterm rupture of the membranes, as recently, it has been suggested
that term and preterm human FMs may have distinct biomechanical
properties. In a recent study, Bhunia et al. [61] compared the
mechanical properties of preterm (33–36 weeks) and term (
One of the reasons for studying biomolecular processes in stretched FM is to find a way to prevent rupture. Barrett et al. [62] developed a mechanical model that mimics the repetitive stretching of the Amniotic Membrane. The authors examined the effect of Cyclic Tensile Strain (CTS) on mediators involved in mechano-transduction Connexin 43 (Cx43) and Protein Kinase B (AKT), tissue remodeling (GAGs, elastin, collagen) and inflammation Prostaglandin E2 (PGE2), and MMPs. Placental and cervical specimens were collected. CTS increased GAG synthesis, PGE2 release, and MMPs activity, with a concomitant reduction in collagen and elastin content. Using Second-Harmonic Generation (SHG) confocal imaging, the authors documented a reduction in intensity after CTS, with regions of disorganization of collagen fibers dependent on tissue location. Furthermore, the observed rise in Cx43 and AKT protein and gene expression following CTS has been reversed using Cx43 antisense or AKT inhibitor. Targeting Cx43 and AKT was suggested as the mean for preventing strain-induced extracellular matrix damage, promoting tissue remodeling mechanisms in the amniotic membrane. The authors concluded that Cx43 could be a potential therapeutic target to prevent inflammation and preterm premature rupture of the fetal membranes [62].
The potential healing effect of Cx43 was further examined by Costa et al. [63] in traumatized human Amn.
Migration of amniotic mesenchymal cells and myofibroblasts, as well as an
increase in Cx43 and Transforming Growth Factor Beta 1 (TGF
The current review provides insights into the relations between macroscopic irreversible deformations and microscopic rearrangement of collagen fibrils, molecular disconnection and reconnection of bonds between fibrils, as well as expression of different proteins associated with disintegration and rupture of the fetal membrane.
A new model for the mechanical response of collagen tissues, which accounts for irreversible deformation, was discussed. The basic assumption of the model is that the constitutive stress–strain relationship of the individual elements which compose the collagen fibers has a plateau segment, during which an irreversible transformation/deformation occurs. During this irreversible stage, collagen rearrangement occurs within the compact layer at both the microscopic and macroscopic scales.
The appearance of a single-valued threshold strain indicates that the most
probable mechanism is a phase transformation of collagen molecules from an
The activation enthalpy of interfibrillar bonds obtained at this stage matches
the typical enthalpy values of polyvalent ionic bonds, indicating a possible
important role of ions like Ca
The biological activity of strain-induced changes in the FM seems to be
connected to mediators involved in mechano-transduction such as Cx43 and AKT,
tissue remodeling like GAG, inflammation mediators PGE2, MMPs (MMP2, MMP9, MMP13)
and IL-1
These proteins, polysaccharides, and proteases should be considered therapeutic targets to counteract preterm premature rupture of the FM.
Biomechanical and biological activities seem to be place-dependent and potentially time-dependent.
Along with the MGI, this overall knowledge can point to ways to heal or prevent damages (microstructural changes) in the FM, thus reducing the risk for preterm labor, at least in some of the patients.
In the future, the emergence of in vivo-like in vitro models such as Organ-On-Chip (OOC) platforms, which recapitulate in vivo functions and responses, has the potential to significantly propel forward research on the FM, for the development of new therapeutic strategies for preventing preterm births, among other challenges [64].
DS and ES wrote and edited the manuscript. Both contributed to the editorial changes in the manuscript, read and approved the final manuscript and agreed to be accountable for all aspects of the work.
Not applicable.
Not applicable.
This research received no external funding.
Given his roles as Editorial Board member and Guest Editor, Eliezer Shalev had no involvement in the peer-review of this article and has no access to information regarding its peer-review. Full responsibility for the editorial process for this article was delegated to Gustavo Caetano-Anollés and William Konigsberg. The authors declare no conflict of interest.
Publisher’s Note: IMR Press stays neutral with regard to jurisdictional claims in published maps and institutional affiliations.