- Academic Editor
Background: Next-generation 5G communication technology involves
increasing use of 3–100 GHz wireless bands in population centers. Though still
non-ionizing, this implies higher radiation energy vs. existing bands. The range
is also shorter, needing more numerous emitters, closer to the user—resulting
in higher electromagnetic energy exposure. With no universal consensus regarding
exposure risks, there is some concern among the public and the scientific
community, following indications that 5G radiation can impact immune function,
trigger inflammatory responses, and influence expression of genes affecting
protein folding, oxidative stress, tissue/extracellular matrix (ECM) matrix
turnover, and more. This work aims at identifying botanical extracts for
protection of human skin from these impacts, based on a preliminary cell
culture-based model. Methods: We irradiated human epidermal
keratinocytes at 6 GHz, evaluating effects on Interleukin1-
5G is shorthand for the incoming fifth generation technology standard for cellular wireless communication networks, whose deployment started worldwide ca. 2019. 5G uses electromagnetic, non-ionizing radiation in several frequency ranges from under 6 GHz and up to 100 GHz [1]. The use of higher frequency bands allows for wider data transfer volumes and higher transfer speeds (more “broadband” communication). 5G technology has thus been built on the use of millimeter waves, significantly shorter than what was used by previous cellular communication generations. As one of the results of this change, 5G has a shorter effective range, and therefore the technology requires a higher number of smaller cells, with a higher number of emitter stations [2]. These emitters would be placed closer together compared to the architecture of previous generations, and necessarily also closer to the end user (up to tens or hundreds of meters [3]). The creation of a ubiquitous network composed of numerous 5G emitters in close proximity to the general population will naturally lead to an increase in exposure to artificial electromagnetic radiation. While the radiation involved remains non-ionizing, there still exists some concern in the general public that the exposure to higher energies may lead to adverse health effects.
While a detailed discussion of the regulatory field is out of scope here, it
should be noted that exposure limits are unclear, and sources (including
regulatory/advisory bodies) have yet to reach any overall alignment and range
from 1 to 20 mW/cm
Further, at the biological level a definite consensus is also still difficult to
identify among the scientific community regarding possible hazards of exposure to
this range of Electromagnetic radiation (EM radiation) [7, 8]. As a starting
point, the very fact that millimeter waves have been used for therapeutic
purposes indicates at least the potential for interactions between these waves
and biological tissue [9]. Several studies seem to suggest that there may in fact
be potential adverse effects [10, 11, 12, 13]. Investigating the effect of millimeter
waves on rat dermis, Millenbaugh et al. [11] showed aggregation of
neutrophils in vessels, degeneration of stromal cells, and breakdown of collagen,
and observed changes in up to 58 genes at 24 h, including genes associated with
regulation of transcription, protein folding, oxidative stress, immune response
including chemokine activity, and tissue matrix homeostasis including
extracellular matrix structure. Real-time reverse transcriptase-polymerase chain
reaction (RT-PCR) confirmed up-regulation of Hspa1a, Timp1, S100a9,
CCL2, and Angptl4. According to these authors, these results indicate
that prolonged exposure to high-frequency millimeter waves “causes thermally
related stress and injury in skin while triggering repair processes involving
inflammation and tissue matrix recovery”. Based on a study on HaCat
keratinocytes, Le Pogam et al. [13] suggested that millimeter waves
affect biomembrane permeability, and showed drastic changes in the extracellular
metabolomic profile. Szabo et al. [12] suggested that exposure of human
skin to millimeter waves can trigger the activation of basal keratinocytes (a key
component of the epidermis), resulting in elevated Interleukin1-
In recent years, considerable dermatological and cosmetic research has been devoted to the (negative) effects of skin exposure to various parts of the electromagnetic spectrum, from Ultraviolet (UV) and high-energy visible (blue) light, and down to infrared [20, 21, 22, 23]. In light of the above, we believe there is a need to expand this focus to include the investigation of the effects of 5G radiation on skin, with a view to helping anticipate the needs of an increasingly exposed population. More specifically, in light discussion above, we believe that the identification of substances capable of protecting human skin from any negative effects of 5G radiation would be a valuable contribution to the field—this was therefore the main ultimate goal this work.
We thus report here on a preliminary in-vitro cell-based model,
exposing a keratinocyte culture to 5G radiation (at 6 GHz) over relatively short
time scales, and on the effects of this exposure on the cell culture. The
endpoints examined, selected based on the precedents cited above and so as to
represent a relevant set of tissue damage indicators, included: IL1-
In pursuit of that specific goal, we also report on the use of the preliminary model described here for the identification of substances able to mitigate the effects of 5G irradiation, e.g., for use as active materials in topical formulations. Marine and terrestrial plant and bacterial extracts have been used to protect the skin from a wide range of stressors, including various parts of the electromagnetic spectrum [30, 31, 32, 33, 34, 35]. This protection effect has often been attributed to anti-oxidative and anti-inflammatory properties of these plant extracts [36, 37]. Following these precedents, we report here on the protective effects observed with three botanical extracts using this same model: an aqueous extract of the aerial parts of Cistus incanus, a Mediterranean shrub rich in polyphenolic metabolites (notably flavanols, including the myricetin glycoside myricitrin), and with demonstrated anti-inflammatory and antioxidant properties, inter alia; an extract of the flower of Trifolium pratense (clover), a widespread medicinal herb also known to possess anti-inflammatory and anti-oxidant properties, containing the flavonoid biochanin A; and an extract of the extremophile Alteromonas sp., whose major component is a soluble exopolysaccharide and which was shown to enhance keratinocyte renewal in vitro [38, 39, 40, 41, 42, 43, 44, 45, 46, 47].
Primary Normal Human Epidermal Keratinocytes (NHEK) (Promocell C-12001,
Heidelberg, Germany; these cells are tested by the supplier for cell morphology,
adherence rate, and cell viability. Immunohistochemical tests for specific
cytokeratins are carried out for each lot. Growth performance is tested through
multiple passages up to 15 population doublings (PD) under culture conditions
without antibiotics or antimycotics. Finally, cells are tested for the absence of
HIV-1, HIV-2, HBV, HCV, HTLV-1, HTLV-2, fungi, bacteria, and mycoplasma.) were
cultured at 37 °C in keratinocyte basal medium (Promocell C-20216,
Heidelberg, Germany) supplemented with 0.06 mM Ca
The cells were blocked with PBS (Sigma Aldrich P3813-10PAK, St Louis, MO, USA)
containing 0.001% Tween-20 (Sigma Aldrich P1379, St Louis, MO, USA), 1% BSA
(Sigma Aldrich A9085, St Louis, MO, USA) and 10% goat serum (Sigma Aldrich,
G9023, St. Louis, MO, USA) during 1 h and then incubated overnight at 4
°C with: anti-TIMP1 mouse monoclonal antibody (Invitrogen MA1-773,
Waltham, MA, USA, diluted 1/2000 in blocking solution); anti-ANGPTL4 rabbit
monoclonal antibody (Invitrogen 701155, Waltham, MA, USA; diluted to 1/500 in
blocking solution); or anti-S100A9 rabbit polyclonal antibody (Invitrogen
PA5-79949, Waltham, MA, USA; diluted to 1/500 in blocking solution). Secondary
antibodies (AlexaFluor® AF488 and AF594, Thermo Fisher, Waltham,
MA, USA) were used for immunofluorescence staining, which was assessed by
microscopical observation. Manual scaling was carried out per field, taking into
account all cells in the field. IL1-
Data analysis was performed using GraphPad Prism 9 (GraphPad Software, Inc., Boston, MA, USA). Statistical tests were selected on the basis of normality and lognormality tests: a parametric test was applied when the normality was positive, and a non-parametric test was used when the normality was negative. For this study, an unpaired Student t-test was applied. Data in the figures is shown as mean +/- Standard Deviation, with p-values appearing on brackets.
Several botanical extracts were used in demonstrating possible protective effects against the effects of 5G radiation, including:
Aqueous extract of Cistus incanus aerial parts
(IBR-Chill™, IFF—Lucas Meyer Cosmetics, Yavne, Israel): Aerial
parts of Cistus incanus were harvested by hand in Southern Israel (by IFF—Lucas
Meyer Cosmetics staff members) [38]. The dried and ground biomass was then
extracted in heated water. Solids were separated, and the resulting liquid was
filtered, yielding a crude extract which was diluted 1:1 in glycerin (Interaxion
C50380681, Deyme, France) and preserved (with 1% Sharomix HP, Sharon
Laboratories, Ashdod, Israel). The final extract’s color is light brown to brown,
with a pH of 4.5–6.5. The extract’s content of the flavonoid glycoside
myricitrin was titrated by high-performance liquid chromatography (HPLC)
(Agilent, Santa Clara, CA, USA: 1100 series with G1315A diode array detector and
Poroshell Phenyl Hexyl 150
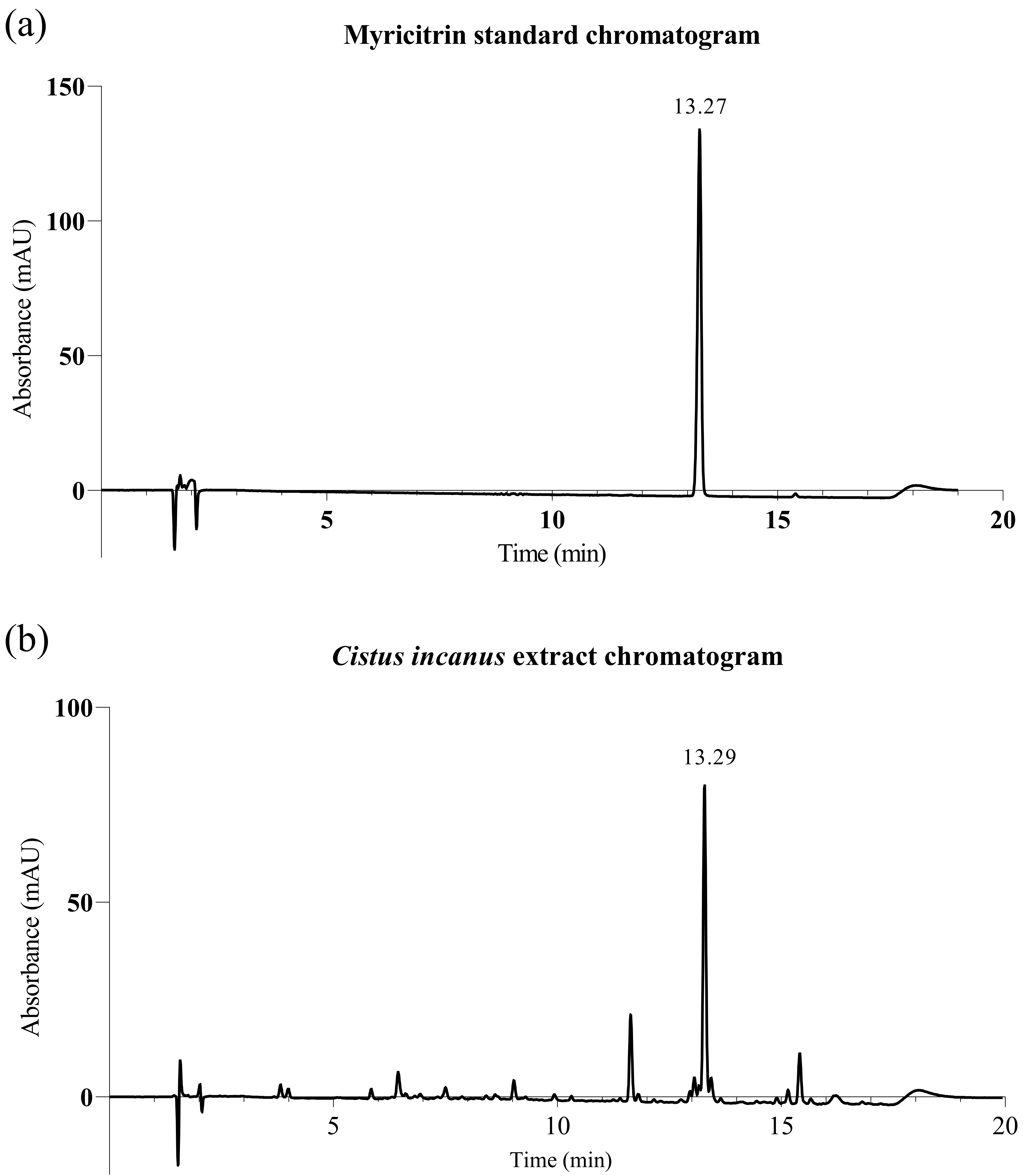
High-performance liquid chromatography with diode-array detection (HPLC-DAD) traces for Cistus incanus extract. (a) Myricitrin standard; (b) Cistus incanus extract, showing the myricitrin peak (ca. 13.3 min) (detection at 355 nm).
Trifolium pratense (Clover) extract (Miniporyl™, IFF –
Lucas Meyer Cosmetics, Toulouse, France): aerial parts of flowering red clover
were harvested in China (Gansu Province), in full accordance with relevant local
regulations. The biomass was extracted in water and ethanol, and the resulting
extract was concentrated and spray-dried. After sieving, isopentyldiol was added,
and the resulting solution was filtered. The final extract is a transparent
liquid, with a pH of 4–8. The extract’s content of Biochanin A was titrated by
reverse phase HPLC chromatography (Agilent, Santa Clara, CA, USA, 1290 series
equipped with Agilent ZORBAX Eclipse Plus C18 2.1
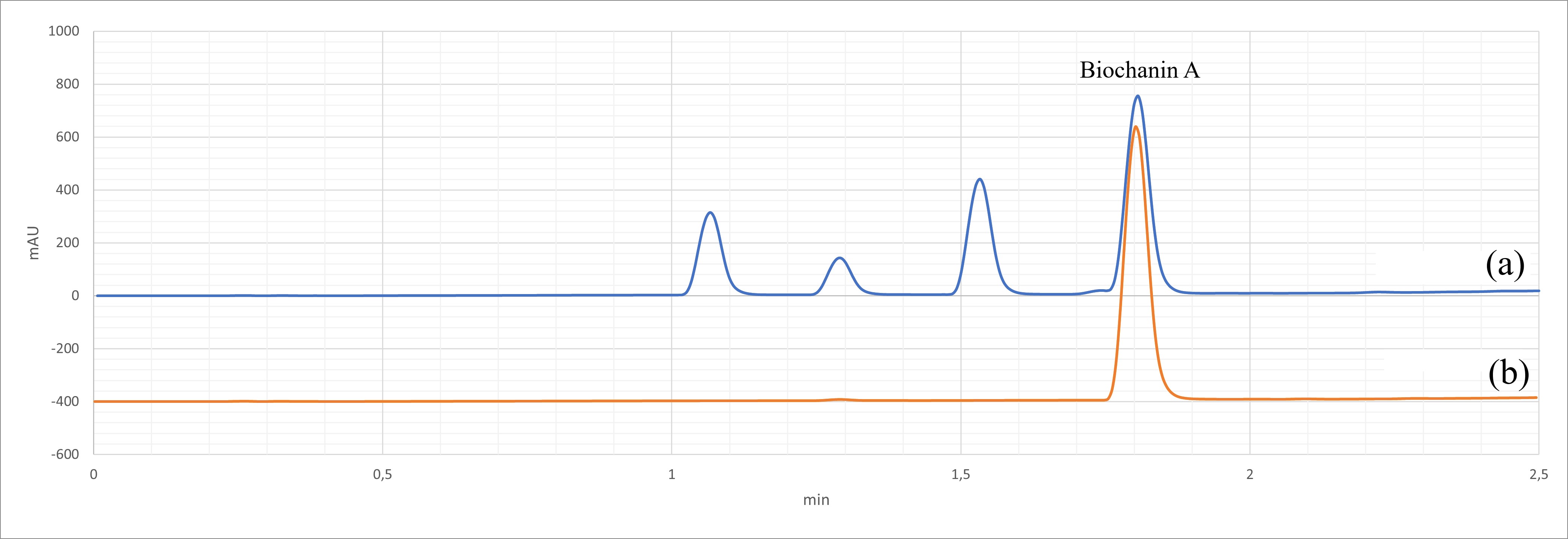
HPLC-DAD traces for Trifolium pratense extract. (a) Trifolium pratense extract, showing the biochanin A peak (ca. 1.8 min); (b) Biochanin A standard (detection at 260 nm).
Alteromonas ferment extract: The extremophile Alteromonas macleodii (subsp. fijiensis), originally isolated from deep-sea hydrothermal vents in proximity to the Galapagos islands, was fermented under conditions propitious to the excretion of a soluble exopolysaccharide (EPS). The ferment was centrifuged and filtered, following which the EPS was precipitated with ethanol, washed, and dried, yielding white cottony fibers. This EPS consists of a repetitive unit of 11 glycosidic residues containing 58% neutral sugars (glucose, galactose, fucose, rhamnose, and mannose) and 30% uronic acids (glucuronic and galacturonic acids). The EPS is finally taken up at 3.0 g/L in water and butylene glycol, resulting in a colorless, slightly viscous aqueous solution, with a pH of 4.70–6.70.
Primary NHEK (Promocell C-12003, Heidelberg, Germany; These cells are tested by
the supplier for cell morphology, adherence rate, and cell viability.
Immunohistochemical tests for specific cytokeratins are carried out for each lot.
Growth performance is tested through multiple passages up to 15 population
doublings (PD) under culture conditions without antibiotics or antimycotics.
Finally, cells are tested for the absence of HIV-1, HIV-2, HBV, HCV, HTLV-1,
HTLV-2, fungi, bacteria, and mycoplasma.) were cultured at 37 °C in
keratinocyte basal medium (Promocell C-20216, Heidelberg, Germany) supplemented
with 0.06 M Ca
The tested products were added in culture medium at 0.1% (Cistus incanus extract and Alteromonas ferment extract) or 0.2% (Trifolium pratense extract) on day 1 (D1), 24 h before 5G exposure, and on D2 (just before 5G exposure). The control cultures did not receive any treatment, except for renewal of the culture medium on the same treatment days.
NHEK were exposed to 5G radiation using a Vector Signal Generator MG3710E
(Anristsu, Atsugi, Kanagawa, Japan), using the following parameters: frequency: 6
GHz; output power; 15 dBm; irradiance: 20 mW/cm
Culture media were collected and stored at –20 °C pending biochemical
assay. IL1-
Data analysis was performed using GraphPad Prism 9 (GraphPad Software, Inc.,
Boston, MA, USA). Statistical tests were selected on the basis of normality and
lognormality tests: a parametric test was applied when the normality was
positive, and a non-parametric test was used when the normality was negative. For
this study, a one-way ANOVA analysis was carried out, followed by
Holm-Šídák’s multiple comparisons test. Data in the figures is shown
as mean
When primary Normal Human Epidermal Keratinocytes (NHEK) were exposed to 5G
bandwidth radiation, clear effects of 5G radiation on the culture of human
epidermal keratinocytes were observable after a remarkably short 1-h exposure
time, in several indicators of keratinocyte function (Figs. 3,4), including: a
strong reduction in collagenase inhibitor TIMP1; a strong increase in wound
healing and epidermal differentiation facilitator ANGPLT4; a marked increase in
S100A9, involved in immune recruitment during injury; and, an increase in key
inflammatory cytokine IL1-
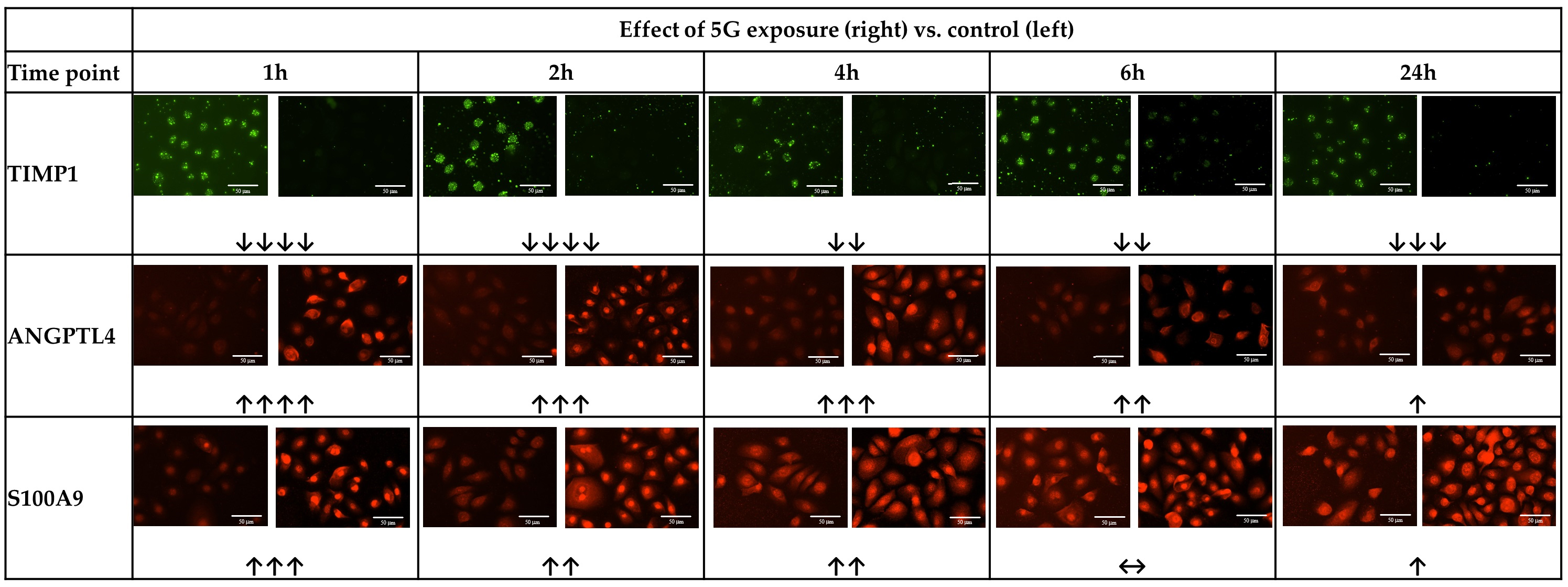
Representative images of immunofluorescence staining revealing
effects of 5G exposure on keratinocytes. Typical images of staining for TIMP
metallopeptidase inhibitor 1 (TIMP1), Angiopoietin-like protein 4 (ANGPLT4), and
S100 calcium-binding protein A9 (S100A9), in a culture of normal human dermal
keratinocytes exposed to 5G radiation for 1–24 h. For each timepoint, comparing
unirradiated control (Left) with 5G irradiation for the time indicated (Right). Scale bar = 50 μm.
Trend evaluation (visual) symbol key: Decrease:
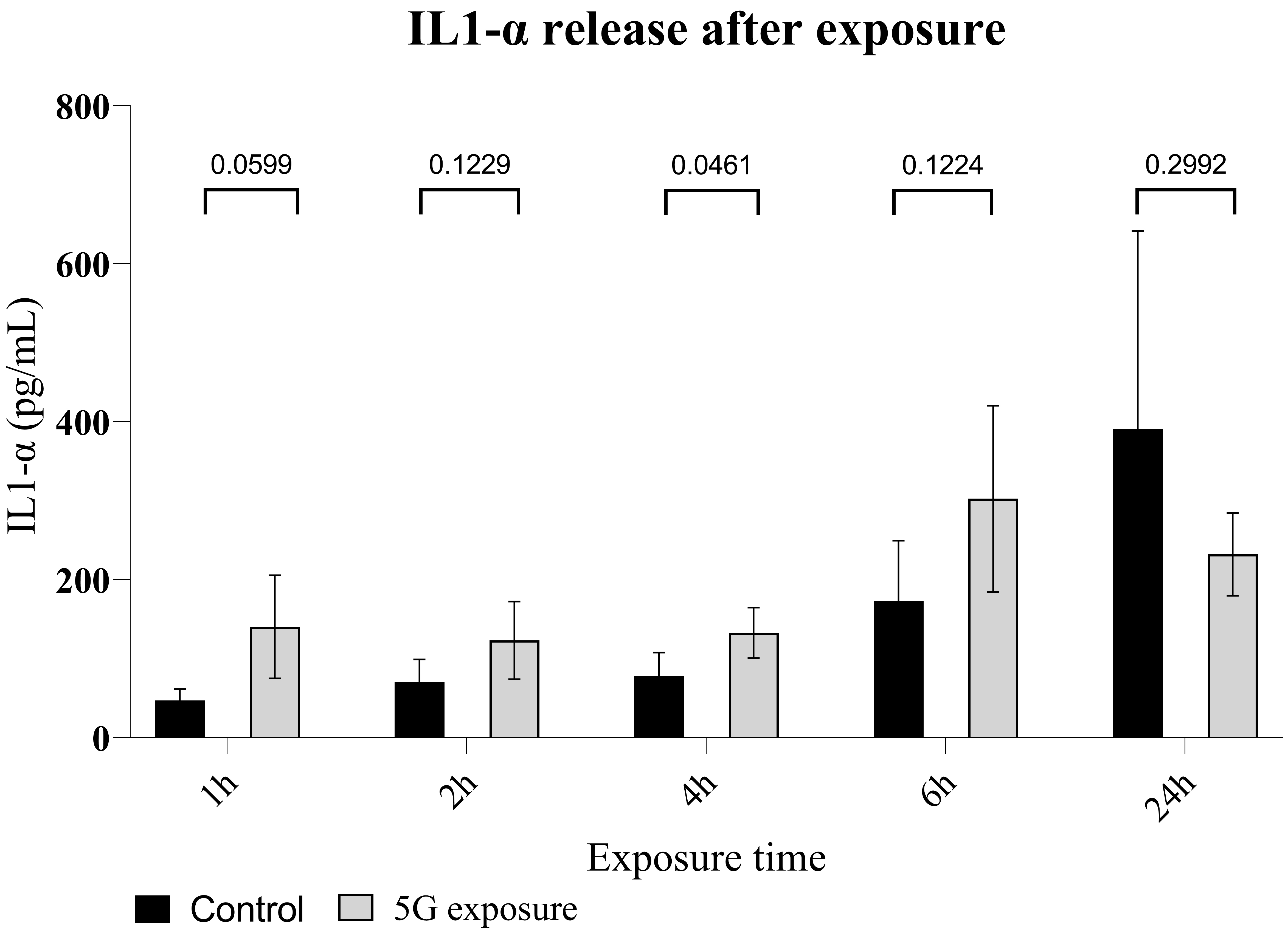
Interleukin 1-alpha release in a culture of normal human dermal
keratinocytes exposed to 5G radiation. Enzyme-linked immunosorbent
assay (ELISA) measurement of IL1-
Interestingly, the effects on all four markers became less marked with longer
exposure times (although, generally, some effect was still detectable). The TIMP1
reduction became less intense from 4 h exposure, though it remained noticeable.
The increase in ANGPTL4 weakened steadily with increasing exposure time, until at
24 h there was only a slight increase vs. the unexposed control. The increase in
S100A9 similarly weakened with increased exposure duration, until there was no
noticeable change at 6 h exposure vs. unexposed control, and only a slight change
at 24 h exposure. The increase in IL1-
Using the same exposure model, we exposed epidermal keratinocyte cultures to 5G
radiation following 24 h pre-treatment with one of several botanical extracts,
including: a Cistus incanus extract, a Trifolium pratense
extract, and an Alteromonas ferment extract (in addition to an untreated
control leg) (Fig. 5). Here again, after 1h of 5G irradiation, the data show a clear
increase in IL1-
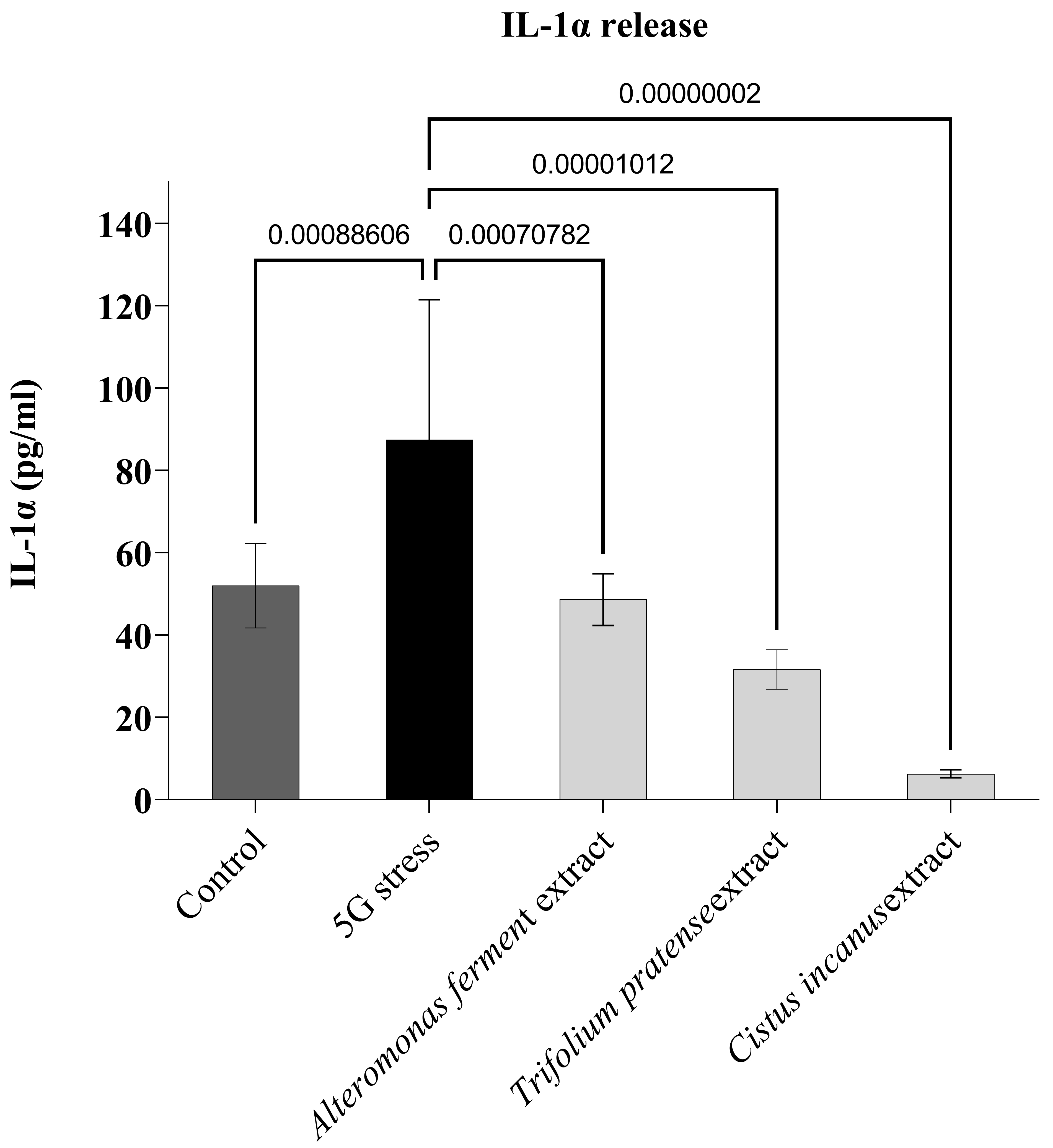
Protective effect of three botanical extracts.
IL1-
Although the results presented above (especially the results of the
immunofluorescence stainings) are mostly qualitative indications, it appears
that, after a short exposure time to 5G radiation (1 h), a (negative) influence
of this radiation on the exposed epidermal keratinocyte culture can be observed.
This includes indications of elevated inflammation status (IL1-
The effects observed with longer, continuous exposure times are somewhat more
ambiguous. Indeed, with continued exposure the effects described above seemed to
weaken considerably—although in most cases they did not disappear entirely. One
could suggest as a possible explanation for these effects the idea that, beyond a
relatively short initial shock, represented by the data collected after 1 h 5G
radiation exposure, we in fact observing injury response adaptive mechanisms
coming into action to mitigate the damage resulting from this exposure, and seek
to restore homeostasis. It cannot, of course, be ruled out that other effects may
also be in play; the observed increase in baseline IL1-
In follow-up studies, it could be very valuable to confirm these directions by increasing exposure times beyond the 24-h mark, with the aim of elucidating whether the cell culture is indeed capable of mitigating the damage caused beyond an initial short-term shock. Further, should this turn out to be the case, a study examining the effect of repeated short-term exposures, seeking to determine whether this type of exposure results in accumulated damage (or, conversely, whether homeostasis mechanisms come into play here also), could be of very high relevance to the continued investigation of the real-world effects of chronic exposure of the population to 5G emissions. Within the bounds of possibility, these studies should use quantitative measures of the effects observed. Finally, additional irradiation parameters such as frequency, intensity, and related others, should be investigated so as to expand the relevance and applicability of this work.
It should be noted that mechanisms such as inflammation and protease activation, both of which our results to be possibly activated by 5G radiation exposure, are widely recognized to lead to premature skin aging, notably via extracellular matrix (ECM) degradation [48]. It thus seems possible that extended and/or chronic exposure to 5G radiation may lead to the premature appearance or worsening of signs of skin aging such as wrinkles, loss of elasticity, pigmentation irregularities, and more.
As shown above, when we pre-treated our keratinocyte culture with each one of
the three botanical extracts presented here, we observed a significantly reduced
increase in IL1-
The effects of EM/radiofrequency radiation are largely recognized as proceeding
at least in significant part via the increase of oxidative stress (possibly
through the generation of Reactive Oxidative Species (ROS)) and the triggering of
inflammatory states in living tissue [8, 49]. In our own work, as described
above, 5G irradiation seems to trigger inflammatory processes in the keratinocyte
culture (as evidenced by increased IL1-
The protective effects observed here may thus be considered quite logical given
some of the extracts’ known composition and biological properties. The
Cistus incanus extract, which showed the strongest protective effect in
our work, has been shown to possess strong anti-inflammatory effects, mediated at
least in part via NF-kB [38]. Cistus incanus extracts have also been
shown to contain polyphenolic compounds (including myricitrin) and possess
antioxidant properties [39]. The latter have also been shown in vivo
with aqueous extracts of the aerial parts of this plant, possibly attributable to
their high polyphenol content [51]. It seems likely that antioxidant properties
also participate in the observed protective effect. The same logic applies to the
Trifolium pratense flower extract, which possesses documented
antioxidant and anti-inflammatory properties, due at least in part to the
presence of flavonoids such as biochanin A [40, 41, 42, 43, 44, 45, 46, 47, 48, 49, 50, 51, 52, 53]. For its part, the
Alteromonas ferment extract has been shown to enhance keratinocyte
renewal in-vitro, and while the exact mechanisms in play have not been
completely elucidated, it is possible that they are linked, directly or
indirectly, to the effect we observe here [47]. Some Alteromonas ferment
extracts have also been shown to possess antioxidant and anti-inflammatory
properties [52, 53]. Finally, it is notable that
The specificity of the effect of the extracts tested here is not yet clear, and
may vary from extract to extract. In the case of the Cistus incanus
extract, which reduced IL1-
In follow-up studies, it would likely be advisable to improve the repeatability of this model, seeking to overcome some of the variability observed between our two studies. The consistency of the primary cells used in the cultures could be a relevant area of focus, for instance—or conversely, it could be interesting to explore the effects of this irradiation on cells from different provenances (e.g., covering a range of donor ages, among other individual characteristics which could influence the results), as well as on additional skin cell types. It may also be valuable to explore the effect of these extracts, and possibly others, on other processes and biological markers which have been shown, here and elsewhere, to be impacted by exposure to 5G radiation. Also, as noted above, it would be interesting to evaluate the protective effect of these and other botanical extracts on a keratinocyte culture exposed to 5G radiation for longer durations (up to, and beyond, 24 h), as well as for short but repeated durations. Additional irradiation parameters such as frequency and intensity should be varied in the context of treatment with botanical extracts, in order to demonstrate protective effects over a wider range of real-life conditions. As suggested by Kostoff et al. [17], it may be useful to examine signal pulsation and modulation, to more closely mimic actual exposure.
The results presented above represent a positive indication as to the potential value of this preliminary cell-based model as a tool for the identification of substances capable of protecting human skin tissue from the effects of 5G radiation, as well as for the in-vitro evaluation of these same effects and the mechanisms involved.
Our data indicate a clear influence of 5G irradiation on the keratinocytes, suggesting induced changes in skin homeostasis which may be consistent with a state of injury and damage response. This appears to corroborate published findings as discussed above, and supports the notion that 5G irradiation may have deleterious effects on the health, and therefore also the appearance, of human skin—possibly, with extended chronic use, leading to premature skin aging and/or the early appearance or worsening of aging signs such as wrinkles or pigmentation irregularities.
Most importantly for the ultimate purposes of this work, the results discussed above demonstrate the potential value of three botanical extracts (extracts of Cistus incanus aerial parts; Trifolium pratense flowers; and Alteromonas ferment) as active ingredients in topical formulations intended to offer protection of human skin from 5G radiation. Best results were obtained with the Cistus incanus extract, likely proceeding via anti-inflammatory and/or anti-oxidant mechanisms.
In future studies, it may be possible to explore these effects in more depth, exploring both the mechanisms involved in the effects of 5G radiation on human skin and the protective effects to be obtained from the use of these and other botanical extracts, with the goal of providing increasingly effective solutions for daily users of 5G technology.
The datasets used and/or analyzed during the current study are available from the corresponding author on reasonable request.
MC, SK, FH, and JA conceptualized and designed the studies presented here; MC and FH were responsible for study execution and data acquisition; MC performed data analysis, curation, and visualization; FH and MC were responsible for data interpretation; JA provided funding and resources, and was responsible for overall project supervision; FH wrote the manuscript, and FH, SK, MC, and JA contributed to its review and edition. All authors have read and approved the present manuscript. All authors have participated sufficiently in the work to take public responsibility for appropriate portions of the content and agreed to be accountable for all aspects of the work in ensuring that questions related to its accuracy or integrity.
Aerial parts of Trifolium pratense and Cistus incanus were harvested in full observance of respective local regulations, by or on behalf of IFF Ltd. - Lucas Meyer Cosmetics. Not relevant for Alteromonas, produced as a microbial culture.
The authors wish to thank their colleagues at IFF-LMC for support with background information and advice on data processing.
This research was funded by International Flavors and Fragrances (IFF—Lucas Meyer Cosmetics), and received no external funding.
The authors are employees of International Flavors and Fragrances and its affiliates, and declare no conflict of interest. Beyond funding our work, the company as such had no role in the design of the study; in the collection, analyses, or interpretation of data; in the writing or editing of the manuscript; or in the decision to publish the results.
Publisher’s Note: IMR Press stays neutral with regard to jurisdictional claims in published maps and institutional affiliations.