- Academic Editor
Background: In this study, we analyzed intestinal
flora in an experimental mouse model of chronic kidney disease (CKD) and
investigated whether oral supplementation with probiotic Lactobacillus
rhamnosus GG could slow the decline in renal function and inflammatory status of
mice with CKD. Methods: We surgically induced chronic
kidney disease in C57BL/6J male mice aged 8–9 weeks. We used dual-stage 5/6
nephrectomy for this, while the mock group underwent a mock procedure. The
experimental (CKD mice) and mock group were administered a daily dose of 10
Chronic kidney disease (CKD) is a condition that progressively affects kidney functions and necessitates renal replacement treatment (dialysis or transplantation). However, it is difficult to assess the precise rate and prevalence of CKD because, in the early to moderate stages, CKD is asymptomatic. The global prevalence of CKD in the general population is about 14%. The worldwide prevalence of CKD was estimated to be about 9.1% in 2017 [1].
Intestinal microbiota is one of the sources of human genetic and metabolic diversity; they encode specific genes totaling over 3 million, which is 150 times more than the number of human genome coding genes, and consequently, they are often termed “the second human genome” [1]. The composition of intestinal microbiota varies from person to person but remains balanced in homeostasis [2, 3]. When this balance of intestinal microbiota is disturbed, it results in both gastrointestinal and extra-gastrointestinal disorders [4]. The progression of underlying disorders leads to an imbalance of intestinal microbiota in individuals with obesity, type 2 diabetes mellitus, liver conditions in people with no history of alcohol consumption (non-alcoholic fatty liver disease or NAFLD), digestive disorders such as inflammatory bowel disease, and perhaps, CKD and advanced level renal conditions [5, 6, 7, 8, 9].
According to new research, the development of toxic metabolites in the circulatory system is inextricably linked to the progression of CKD to end stage renal diseases (ESRD) [10]. This buildup may lead to an increase in the dysbiotic microbiome’s generation of toxins and a decrease in their elimination due to the compromised renal function in patients with CKD. Studies indicate that intestinal inflammation and impairment of the epithelial barrier accompany the pathological development of CKD, speeding up the movement of bacteria-derived uremic toxins throughout the body and causing damage to the renal system, cardiovascular, and central nervous systems as a result of oxidative stress [10, 11, 12]. These findings present novel treatment approaches for the control of uremia, inflammatory conditions, and kidney disease development as well as the prevention of negative consequences in patients with CKD. Therefore, patients with CKD may benefit from treating uremic toxins with dietary therapies that include probiotics, prebiotics, and synbiotics.
Recent studies have shown that taking probiotic supplements slows the development of CKD. In this regard, the majority of microorganisms studied in previous research belong to the Bifidobacterium and the Lactobacillus species; positive outcomes included reductions in urea concentration, blood urea nitrogen (BUN), and ammonia levels. This observation was also noted in indoxyl sulfate plasma concentrations of p-cresol [13]. These probiotic strains are also said to drastically reduce levels of cytokines and endotoxins while increasing populations of Bifidobacterium, a genus considered to be essential for the functionality of the intestinal mucosal barrier.
In this study, our objective was to evaluate the impact of administering Lactobacillus rhamnosus GG (LGG) to mice with CKD. We evaluated serum markers of renal injury, nutritional indicators, concentrations of inflammatory factor interleukin 6 (IL-6), and the abundance and biodiversity of stool microbiota in mice with CKD.
We used male C57BL/6J mice as the experimental subjects. The mice were specifically aged 8 to 9 weeks. The mice were acquired from the Second Affiliated Hospital of Harbin Medical University and kept in a room with a controlled 12-hour light-to-dark cycle, 25 °C temperature, and 50% humidity. First, the mice were fed for one week and randomly divided into four groups: (1) sham + placebo control; (2) sham + LGG; (3) CKD + placebo control; (4) CKD + LGG.
10% chloral hydrate was used for abdominal anesthesia before the operation in all groups, and a left dorsal median longitudinal incision was performed to expose the left kidney. In the CKD groups, the capsule was separated, the left renal pedicle was clipped, and the upper and lower 1/3rd renal parenchyma of the left kidney were cut off. The residual kidney was placed back into the abdominal cavity after electrocoagulation and hemostasis. In the sham groups, only the capsule was separated, the renal pedicle was not clipped, and the renal parenchyma was not removed.
One week later, we removed the right kidney of the mice in the CKD groups, while in the sham groups, only the capsule was separated, and the right kidney was not removed. Five weeks after 5/6 nephrectomy removal, we collected blood from the tail vein to detect serum creatinine. The model was deemed successful when the serum creatinine was more than twice the normal standard [12]. The final number of mice included in each group was 8.
Both the CKD-induced mice and the mock mice were administered a daily dose of 10
This study was conducted in accordance with the Guide for the Care and Use of Laboratory Animals (Eighth Edition) issued by the U.S. National Institutes of Health. This study was approved by Harbin Medical University’s Ethics Committee of the Second Affiliated Hospital (No. Ky2020-045).
The experimental subjects were euthanized after a night of starvation marking the end of the study, and blood samples were taken for further examination. We measured the levels of BUN, serum creatinine, cystatin C, albumin, C-reactive protein (CRP), and IL-6, and determined the peripheral white blood cell count. Detailed information on the commercially available test kits that were used is listed in Supplementary Table 1.
The mouse kidneys were dehydrated using graded ethanol after being embedded in 4 percent paraformaldehyde, vitrified by dimethylbenzene, and embedded in paraffin blocks according to an established protocol [15]. The specimens were sliced into continuous 4-µm paraffin sections, which were subject to eosin and hematoxylin staining using fluorescence microscopy.
Tissue samples from the kidneys were scored histopathologically. The degree of
kidney injury was determined by pathological scoring (0–4) as renal tubular
epithelial cell enlargement, mesangial proliferation, glomerular endothelial
swelling, or renal fibrosis. The scoring criteria were as follows: 0 (
At the end of the study period, we obtained samples made of fresh mice feces,
and microbial deoxyribonucleic acid was extracted using the QIAamp fast
deoxyribonucleic acid (DNA) extraction equipment (catalog number 51604).
Polymerase chain reaction (PCR) was used to amplify the V3–V4 zones of the
microbes 16S ribosomal ribonucleic acid genes (rRNA) at 95° for 3 minutes,
followed by 30 cycles at 98° for 20 seconds, 58° for 15 seconds, and 72° for
20 seconds, with a final extension at 72° for 5 minutes using primers 341F
5
Amplicons were obtained from 2 percent agarose or polyacrylamide, refined with an AxyPrep DNA Gel Extraction Kit (Axygen® Biosciences, Union City, CA, USA) (per the package recommendations), and computed with a Qubit® 2.0 fluorometer (Invitrogen, Carlsbad, CA, USA). Following the preparation of the library, the tags sequencing was done using an Illumina NovaSeq PE250 apparatus (San Diego, CA, USA). For the sets of two reads of 250 base pairs, the bps intersected on 3 endpoints for synthesis into the initial lengthier tags. The extraction of DNA, library preparation, and sequencing procedures were carried out at the Realbio Genomics Institute (Shanghai, China) [17, 18].
The tags were examined for rest durations and average base quality after being cleared of primers and barcodes. All 16S tags were limited to 220 to 500 bp, in such a manner that the aggregate Phred value of the bases was not any lower than 20 (Q20) and there was a maximum of only three ambiguous N. The tags’ copy number was counted, and the duplication of repeating tags removed. Only tags with a frequency greater than one were grouped in operationally taxonomic units (OTUs), with each having a characteristic tag.
We used the UPARSE (http://drive5.com/uparse/) program to cluster these OTUs with 97 percent homology, and chimeric sequences were identified and deleted by using USEARCH (version 7.0) software (http://www.drive5.com/). Taking SILVA database as reference (https://www.arb-silva.de/), Ribosomal Database Project (RDP) classifier (http://rdp.cme.msu.edu/) was used to systematically classify the OTU sequence. The classification is based on Bergey’s taxonomy, and the default threshold is 80%. The OTU profile table and alpha-beta diversity studies were carried out in Quantitative Insights Into Microbial Ecology (QIIME) using Python scripts [17, 18].
Data analysis was conducted using the Statistical Package for Social Sciences
(SPSS) system (version 22.0 for Windows, IBM Corp., Armonk, NY, USA). The
standard error of the mean is used to express quantitative data (SEM). We used
the Kruskal-Wallis test (for more than two groups) or the Wilcoxon rank-sum test
(for two groups) to evaluate the variations between the measurable groups of
data. The data was analyzed using a two-way analysis of variance (ANOVA) with
Tukey’s post hoc testing. If the p values for the Kruskal-Wallis tests
were substantial (p
All mice were fed ad libitum during the 5-week study. No difference was observed in initial body mass, final mass, or change of weight among the four groups (Fig. 1A–C). We evaluated the effects of L. rhamnosus GG supplementation on kidney function and found the BUN and serum concentrations of creatinine and cystatin C to be significantly elevated in the mice with CKD (Fig. 1D–F). Importantly, supplementation with L. rhamnosus GG reduced both BUN and serum creatinine in the mice with CKD.
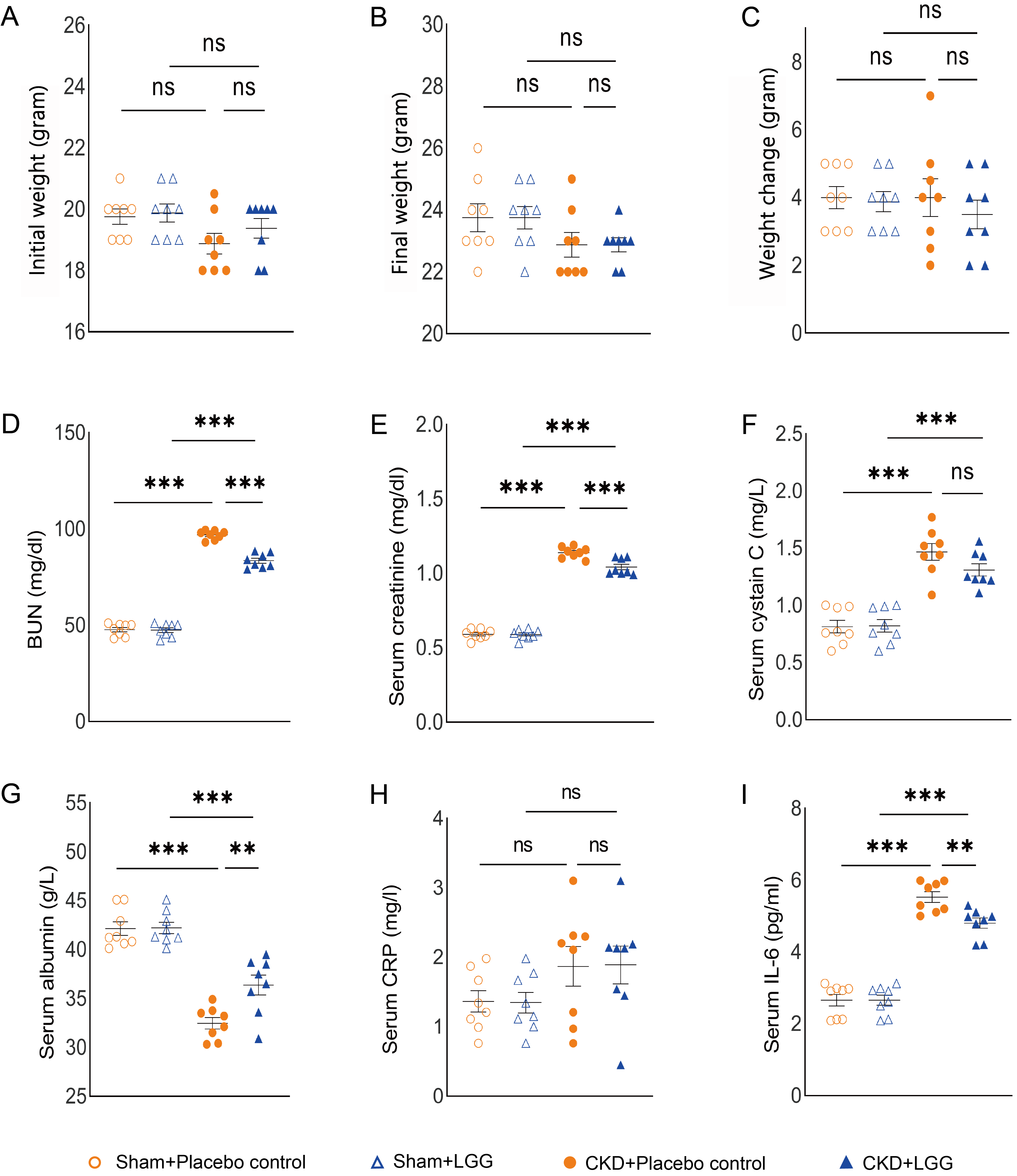
Physical properties and biochemical analysis of 5/6 nephrectomy
mice with chronic kidney disease (CKD) after 5 weeks of treatment. (A) Initial
weight. (B) Final weight. (C) Weight change. (D) Serum blood urea nitrogen
levels. (E) Serum creatinine levels. (F) Serum cystatin C levels. (G) Serum
albumin levels. (H) Serum C-reactive protein levels. (I) Serum interleukin 6
levels. “ns” indicates no significant difference. **p
Previous research found that hypoalbuminemia as well as elevated CRP were major predictors of overall-cause and cardiovascular death in patients with chronic kidney disease [19]. We found that supplementation with L. rhamnosus GG significantly increased the serum concentration of albumin in the mice with CKD, whereas there was no difference in the concentration of serum CRP between the mice with CKD treated with L. rhamnosus GG versus those treated with a placebo (Fig. 1G,H). Supplementation with L. rhamnosus GG significantly decreased the serum concentration of IL-6 in CKD (Fig. 1I).
At the end of the study, renal pathological damage was confirmed in the mice with CKD. Histopathological examinations by hematoxylin and eosin (H&E) staining revealed a significant increase in inflammatory cells in the glomeruli, swollen epithelial cells in the renal tubules, increased inflammatory cells in the renal tubules, granular degeneration of cells, and the presence of epithelial detachment in the renal interstitium (Fig. 2C). Importantly, supplementation with L. rhamnosus GG attenuated renal pathology in CKD (Fig. 2D,E).
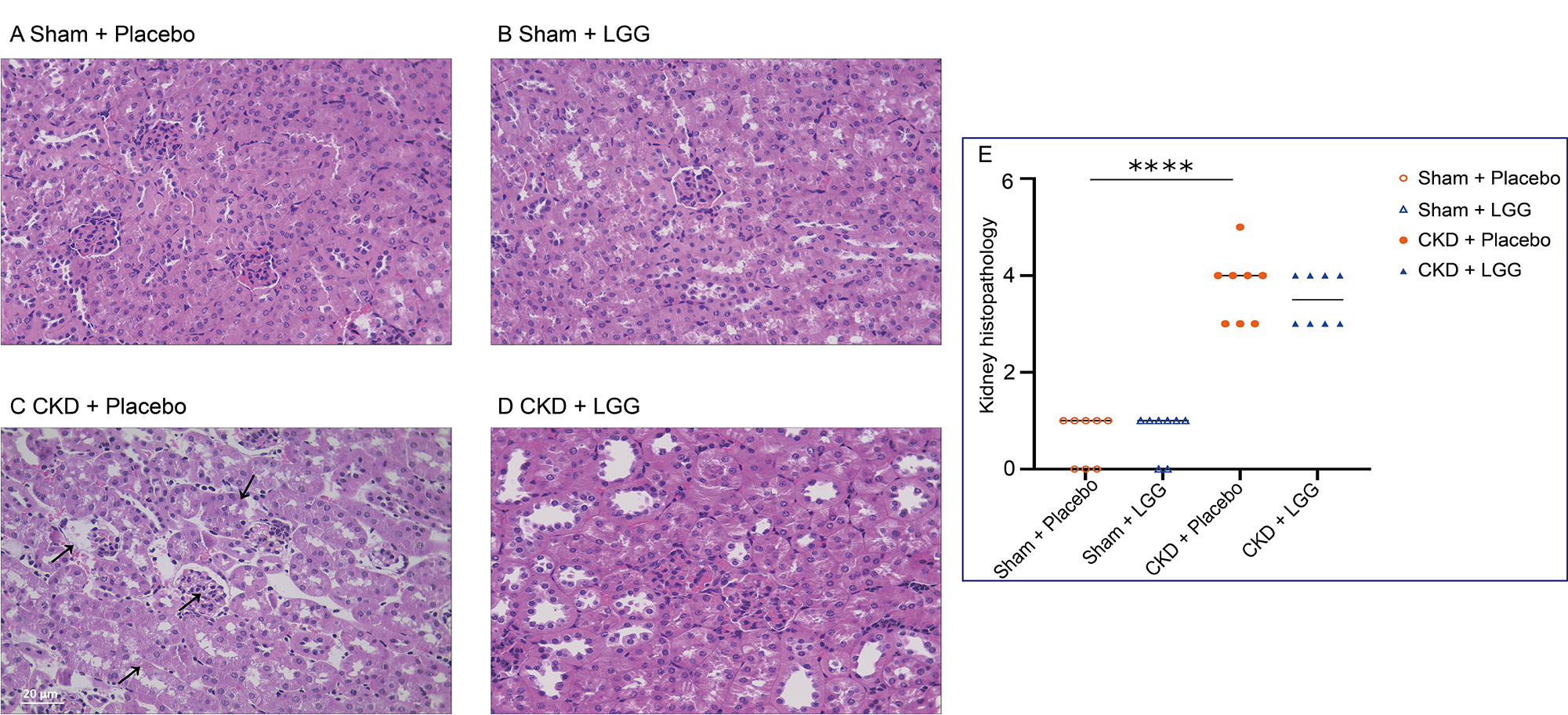
Hematoxylin and eosin-stabilized kidney sections in 5/6
nephrectomy mice with chronic kidney disease. (A) Sham + Placebo group. (B) Sham
+ LGG group. (C) CKD + Placebo group. (D) CKD + LGG group. (E) Renal pathological
score. The severity of each injury was assessed by a 0–4 score: 0 = minor
damage, 1+ = mild damage, 2+ = moderate damage, 3+ = severe damage, and 4+ =
serious damage. “ns” indicates no significant difference. ****p
In comparison with the mock + placebo group, the CKD + placebo had inflammatory cells in the glomeruli, inflamed epithelium in the kidney tubules, granular neurodegeneration, and inflammatory cells inside the interstitium; there was also some epithelial detachment. In the CKD + L. rhamnosus GG (LGG) group, a small number of inflammatory cells were found in the glomeruli, the epithelial cells of the renal tubules were swollen, and granular degeneration was present (Fig. 2).
The renal pathological scores of the sham + placebo and sham + LGG groups were
The freshly isolated fecal samples of the mice were analyzed. One hundred and sixty-seven OTUs were identified in mice with CKD versus mock mice, of which 76 OTUs showing significant differences (Supplementary Table 2). Among them, 24 OTUs such as Lactobacillus, Lactococcus, Bifidobacterium, and Alloprevotella reduced greatly in the CKD + placebo subjects relative to the sham + placebo subjects. Fifty-two OTUs such as Prevotellaceae UCG-001, Lachnospiraceae UCG-001, Lachnospiraceae, and Lachnoclostridium decreased greatly in the mock group relative to the CKD unit. One hundred and sixty-six OTUs were detected in the mice with CKD treated with L. rhamnosus GG versus the mice with CKD treated with a placebo, of which 14 OTUs had significant differences.
Rs-E47 termite, Enterorhabdus, Enterococcus, UCG-010, Ileibacterium, Clostridia, Chrisensenellaceae R-7, Anaerovorax, and Family XIII AD3011 decreased significantly in the CKD + placebo group. Bacteroides, Alistipes, Erysipelatoclostridium, and Clostridium innocuum decreased significantly in the mice with CKD treated with L. rhamnosus GG relative to the sham mice treated with L. rhamnosus GG (Fig. 3A).
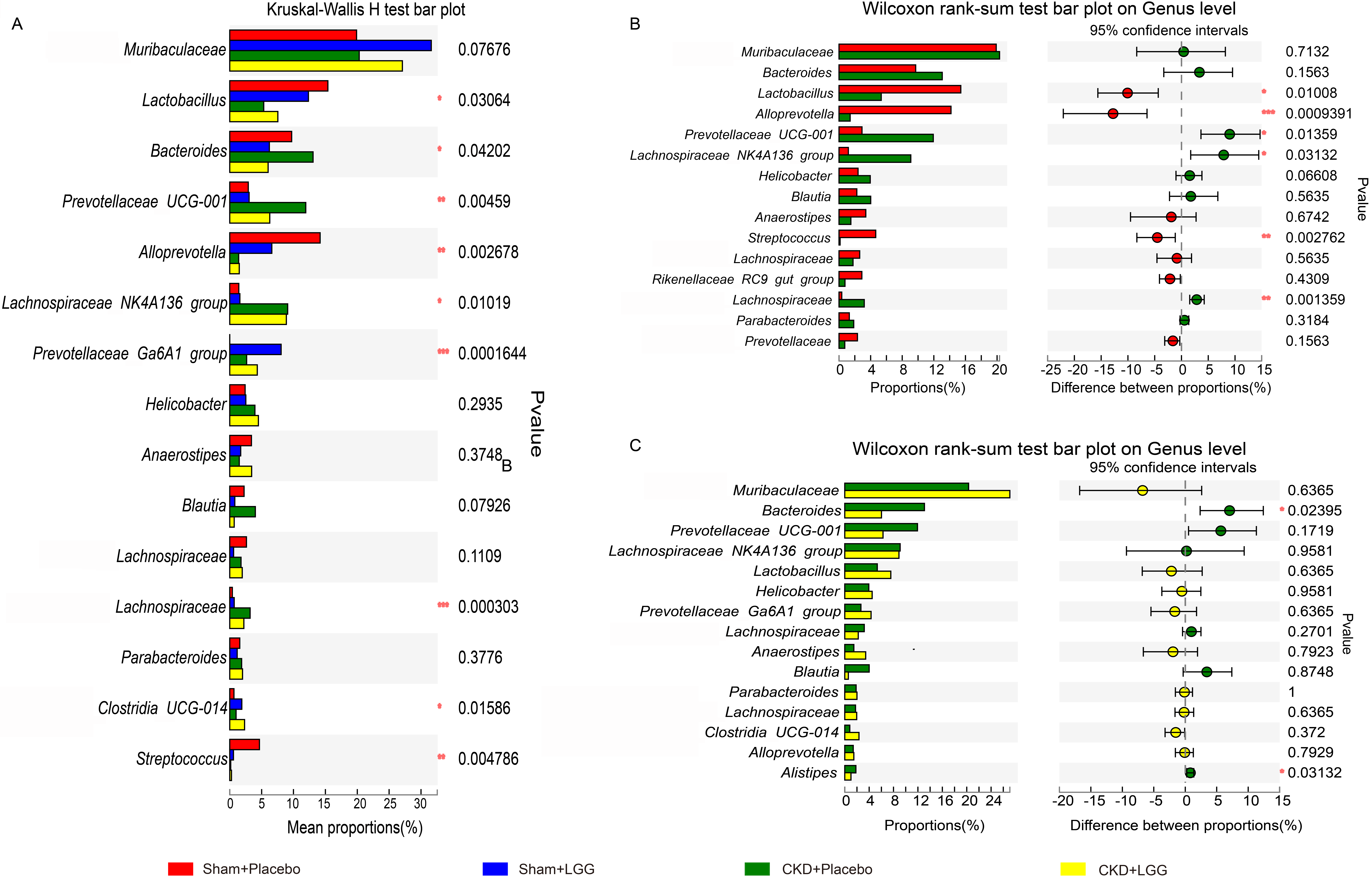
Species diversity analysis. The y-axis represents the species
name at a taxonomic level, the x-axis represents the average relative abundance
in different groups of species, and the columns with different colors represent
different groups; the rightmost column is the p value. (A) Comparison
between the Sham + Placebo, Sham + LGG, CKD + Placebo, and CKD + LGG groups. (B)
Comparison between the sham + Placebo group and the CKD + Placebo group. (C)
Comparison between the CKD + Placebo group and the CKD + LGG group. *p
Furthermore, substantial variations were observed between the placebo-treated CKD mice and the sham mice among the 15 most abundant OTUs, in terms of Lactobacillus, Alloprevotella, Prevotellae UCG-001, Lachnospiraceae-NK4A136, Streptococcus, and Lachnospiraceae (Fig. 3B). Only Alistipes and Bacteroides were different in term of abundance among the 15 most prevalent classes in the CKD mice studied (Fig. 3C). Lactobacillus was the third most dominant OTU, and it decreased significantly in the mice with CKD. In mice with CKD, L. rhamnosus GG administration increased the number of Alloprevotella and Lactobacillus while at the same time decreasing the prevalence of Lachnospiraceae and Prevotellae-UCG-001, Lachnospiraceae-NK4A136.
A coverage index analysis revealed that the sample coverage of the four groups
of mice was
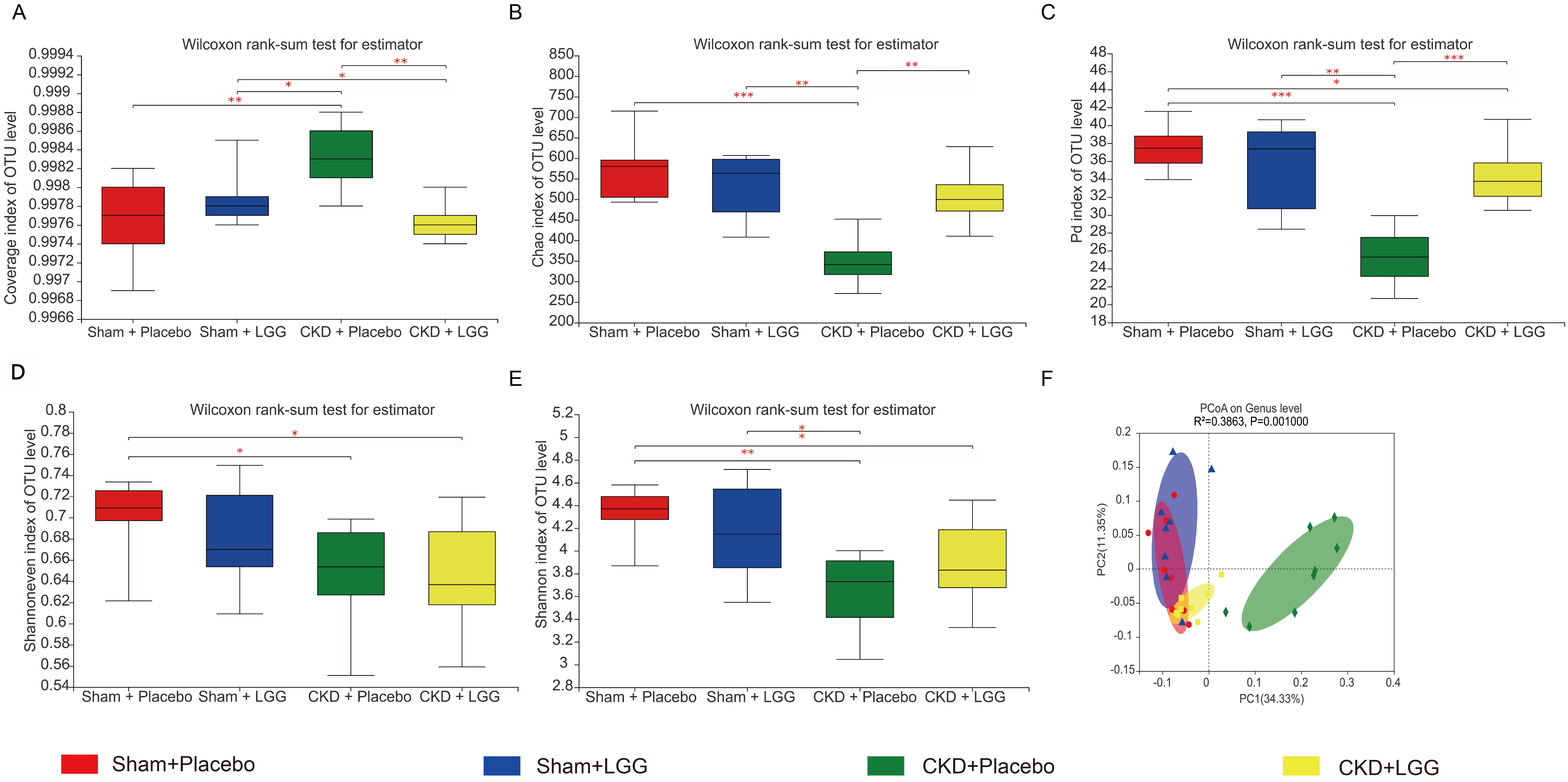
Alpha-diversity and beta-diversity analysis of intestinal
microbiota. The x-axis represents the grouping, and the y-axis represents the
alpha diversity index under different groupings: (A) community coverage index,
(B) species richness Chao1 index, (C) phylogenetic diversity index, (D)
Shannoneven index, (E) Shannon index, and (F) beta-diversity principal
coordinates analysis plot. The x and y axes represent the two selected principal
axes, and the percentage represents the interpretation value of the principal
axis to the difference in sample composition. The scale of the x and y axes is a
relative distance, which has no practical significance. Points with different
colors or shapes represent samples from different groups. The closer the two
sample points, the more similarity between the species composition of the two
samples. *p
In comparison with the CKD-induced group, the community richness of the CKD mice treated with L. rhamnosus GG significantly improved, and there was no difference between the CKD mice treated with L. rhamnosus GG versus the sham mice treated with a placebo, indicating that the community richness of the CKD mice returned to normal after supplementation with L. rhamnosus GG (Fig. 4B).
Phylogenetic diversity (PD) reflects the biodiversity of an entire microbial community. Although the PD of the CKD mice treated with L. rhamnosus GG was substantially more than observed in CKD mice treated with a placebo, the PD of the CKD mice clinically treated with L. rhamnosus GG was much lesser than that of the sham mice treated with a placebo, showing that the PD of the microbial group significantly improved after supplementation with L. rhamnosus GG, although it did not return to normal (Fig. 4C). Our results demonstrate that probiotic supplementation helps restore intestinal microecological disorder.
A beta diversity principal coordinates analysis (PCoA) is a
dimensionality-reduction technique that can be used to evaluate similarities and
differences in test group makeup. According to the PCoA analysis of unweighted
UniFrac, there was aggregation in the four different test groups, indicating that
the genera in the set were similar. However, differences were observed in the
four distinct groups (p = 0.001, R
In this study, we used a linear discriminant analysis effect size (LEfSe) difference analysis for screening the mice. The LDA analysis score in this experiment was 4. Actinobacteria, Firmicutes, and Bacteroidota appeared to be the prevalent phyla in the sham mice, while Bacteroidota and Firmicutes were the leading phyla in the sham mice treated with L. rhamnosus GG. In the CKD mice, Bacteroidota and Firmicutes were the dominating phyla, whereas Firmicutes was the dominating phylum in the CKD mice treated with L. rhamnosus GG.
Although different groups shared the same dominant phyla, the dominant phyla were different. Bifidobacterium are known to be beneficial bacteria, and along with Lactobacillus, they were dominant in the sham mice. L. rhamnosus GG was used to treat the mice that were dominated with Erysipelotrichales and Bacteroidales, while Bacteroidales and Lachnospirales dominated in the CKD mice. CKD mice treated with L. rhamnosus GG exhibited Oscillospirales dominance (Fig. 5).
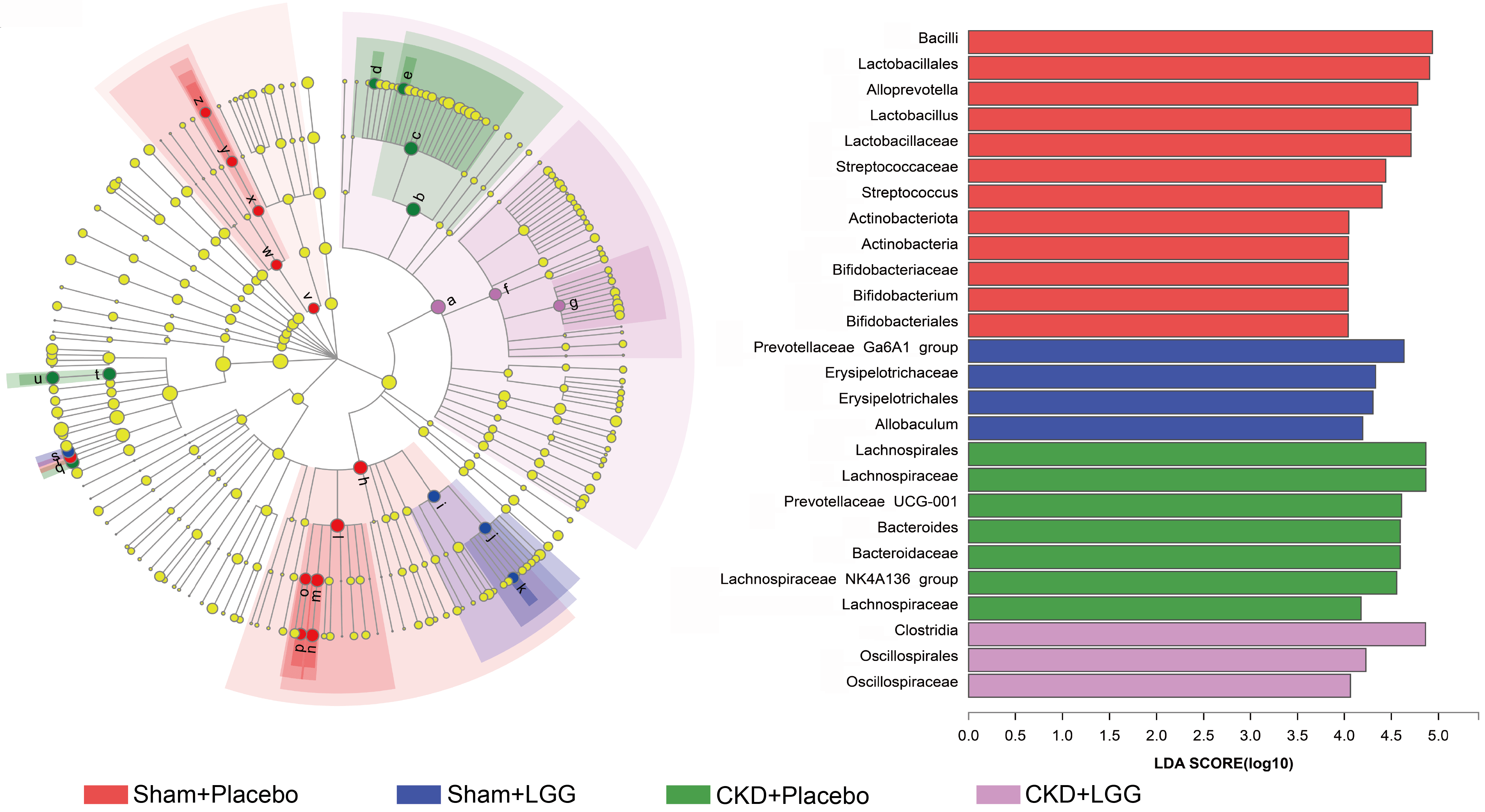
Linear discriminant analysis effect size (LEfSe) multistage species difference discriminant analysis. Different color nodes represent microbial groups that are significantly enriched in the corresponding groups, with a significant impact on the differences between groups. Light-yellow nodes indicate microbial groups with no significant difference in the different groups or have no significant effect on the difference between groups. The x-axis represents the correlation abundance, the y-axis represents the different groups, and different colors represent different functions.
CKD can lead to intestinal microecological disorder via the buildup of uremic toxins present in the intestines, thereby accelerating disease progression [19]. When CKD progresses to ESRD, it can be complicated with other systemic diseases, such as cardiovascular disease, which increases mortality. Intestinal flora and their metabolites participate in physiological functions, such as nutrition, metabolism, and inflammation, in many ways [20, 21]. In this study, we demonstrated that supplementation with the probiotic L. rhamnosus GG improved both renal function and nutritional status, reduced the micro-inflammatory state, and improved intestinal microecology in mice with CKD. In addition, supplementation with L. rhamnosus GG increased the richness and PD of the intestinal flora of the mice. Our findings suggest that L. rhamnosus GG can be a viable treatment to delay the development of CKD.
CKD can increase the expression of skeletal muscle atrophic protein, reduce skeletal muscle synthetic protein, increase fat synthesis, and cause weight loss [22]. In an experiment to study the intestinal flora of CKD in beagles, the administration of different doses of betaine and soluble fiber had no significant effect on the total body mass of the dogs [23]. In our study, all the mice were given free access to food. We found that neither the placebo treatment nor the L. rhamnosus GG treatment had significant effects on the mice’s individual and collective weight (Fig. 1A–C). Although there were insignificant differences in total mass, we believe that the content of lean body mass and total fat may have changed.
CKD reduces renal function, resulting in a decrease in uremic toxin clearance. A large number of uremic toxins accumulate in the circulatory system, increasing the body’s inflammatory response and further deteriorating renal function [24]. In addition to the kidney, the intestine is also an important site for uremic toxin metabolization [25]. Microorganisms in the intestine help the body’s amino acid breakdown; for example, microorganisms can metabolize tryptophan to indole and convert it into indole sulfate in the liver. In our study, we demonstrated that L. rhamnosus GG supplementation decreased BUN and circulation of nitrogen and concentration of serum in creatinine (Fig. 1D,E), indicating that L. rhamnosus GG could attenuate the progression of renal damage.
Inflammatory factors play a key function in CKD. In a mouse model of lupus nephritis [14], increasing the colonization of Lactobacillus decreased the production of IL-6, augmented the production of IL-10 in the intestine, and reduced the micro-inflammatory response in vivo. We demonstrated that the probiotic L. rhamnosus GG significantly reduced serum concentrations of IL-6 in CKD mice (Fig. 1I).
CKD was found to increase the loss of protein in vivo. After supplementation with L. rhamnosus GG, the serum albumin concentration in the mice increased significantly, suggesting that L. rhamnosus GG could improve CKD hypoproteinemia (Fig. 1G).
In our study, the CKD mice developed renal pathological damage. The results of H&E staining revealed that the inflammatory cells in the glomeruli of the CKD mice increased significantly, the epithelial cells in the renal tubules swelled, the inflammatory cells increased, the cell granules degenerated, and the epithelium exfoliated in the renal interstitium. However, renal injury was alleviated after supplementation with L. rhamnosus GG, revealing that the probiotic could delay the progress of renal pathology (Fig. 2).
The results of our coverage index analysis indicated that this study’s sequencing dimensions enclosed all genera in the sample. Further analysis revealed that CKD seriously damaged the stability of the mice’s microecology of the intestines and significantly reduced the uniformity, richness, and diversity of their intestinal microorganisms (Fig. 3). The findings of this study are in line with the analysis of the intestinal flora of rats with CKD by Vaziri et al. [26]. However, when analyzing the intestinal flora of mice with acute renal injury, it was found that renal ischemia–reperfusion did not alter the richness and diversity of the flora [4], suggesting that the length of the course of renal disease had a certain impact on the intestinal microecology of the mice.
In addition, in the PCoA analysis of the four groups of mice, the PCoA scores for the sham + placebo, sham + LGG, CKD + placebo, and CKD + LGG groups were found to be similar within the groups, and the samples of the sham + placebo, sham + LGG, and CKD + LGG groups have overlapping parts in the figure. The centralized trend suggested that the species makeup of the three groups was similar. However, the composition of the CKD mice administered with a placebo was substantially different from the remaining three categories.
Moreover, we found that the dominant intestinal phyla of the sham + placebo, sham + LGG, CKD + placebo, and CKD + LGG groups were similar to Firmicutes, Actinobacteria, and Bacteroidota; however, upon further analysis, the dominant orders of the sham + placebo group were mainly Bifidobacterium and Lactobacillales, while the prevalence of Bifidobacterium and Lactobacillales reduced in the CKD + placebo category. The abundance of Bacteroidales and Lachnospirales increased significantly.
Studies have shown that Lactobacillus inhibits the invasion and growth of Escherichia coli and other pathogens by activating other probiotics in the intestine to compete with pathogens, redistributing intestinal tight-intersection-related proteins, as well as promoting the recovery of the barrier role of the intestinal wall. E. coli can reduce the expression of the tight-junction protein, while Lactobacillus can increase it [11, 12, 27]. In this experimental study, alpha–beta multiplicity analyses of the intestinal microorganisms and a species diversity analysis revealed that supplementation with L. rhamnosus GG could restore disordered intestinal microecology to a certain extent.
In previous studies, the intervention of probiotics on CKD had some similar benefits, such as increasing the counts of Bifidobacterium and Lactobacillus, reducing the formation of uremic toxin, p-cresol and its serum concentration, improving lipid metabolism, and reducing the concentration of systemic inflammatory state and oxidative stress markers [28, 29, 30]. Our research results showed that supplementation of L. rhamnosus GG had similar effects on improving CKD as supplementation of other probiotics, such as reducing the inflammation level and controlling nitrogen production. However, there were some differences in the effect of improving intestinal flora. In our study, the administration of L. rhamnosus GG increased the number of Alloprevotella and Lactobacillus in mice with CKD. This may be because different probiotics have different regulatory preferences. In the future, whether different probiotic interventions have similar effects on improving renal function through common mechanisms remains to be further studied.
We demonstrated that the progression of CKD disturbed intestinal microecology, and this can be an important factor in further aggravating renal injury. Importantly, the use of the probiotic L. rhamnosus GG improved both renal function and nutritional status and restored the intestinal microecology of mice with CKD.
Therefore, supplementation with L. rhamnosus GG could be a useful therapeutic approach to delay the decline of renal function, improve the intestinal microenvironment, and ultimately, improve quality of life and long-term prognosis for patients suffering from CKD and ESRD.
All data generated or analysed during this study are included in this article. Further enquiries can be directed to the corresponding author.
Conception and design of the research: PZ, LZ. Acquisition of data: JN, LX, LYY, YYY, LHS, XRL, YQW. Analysis and interpretation of the data: PZ, LZ. Statistical analysis: JN, LX, LYY, YYY, LHS, XRL, YQW, XCT. Obtaining financing: PZ, LZ. Writing of the manuscript: LZ. Critical revision of the manuscript for intellectual content: PZ, LZ. All authors contributed to editorial changes in the manuscript. All authors read and approved the final manuscript. All authors have participated sufficiently in the work to take public responsibility for appropriate portions of the content and agreed to be accountable for all aspects of the work in ensuring that questions related to its accuracy or integrity.
This study was conducted with approval from the Ethics Committee of the Second Affiliated Hospital of Harbin Medical University (No. Ky2020-045). This study was conducted in accordance with the declaration of Helsinki. All applicable international, national, and/or institutional guidelines for the care and use of animals were followed.
We would like to acknowledge the hard and dedicated work of all the staff that implemented the intervention and evaluation components of the study.
(1) 2022 Key R&D Plan of Science and Technology Department of Sichuan Province (2022YFS0149); (2) Science and Technology Fund of Chengdu Medical College in 2021 (CYZYB21-22); (3) Science and Technology Fund of Chengdu Medical College in 2022 (CYZYB22-08); (4) 2023 Innovation Fund of Sichuan Maternal and Child Health Hospital (CXZD2023-05); (5) 2023 Innovation Fund of Sichuan Maternal and Child Health Hospital (CXZD2023-01); (6) 2021 Sichuan Medical Research Project of Sichuan Medical Association (S20137); (7) Medical Research Project of Chengdu Municipal Health Commission (2022113); (8) “Chunhui Plan” Cooperative Scientific Research Project, Ministry of Education of the People’s Republic of China(HLJ2019023).
The authors declare no conflict of interest.
Publisher’s Note: IMR Press stays neutral with regard to jurisdictional claims in published maps and institutional affiliations.