- Academic Editor
This is an open access article under the CC BY 4.0 license.
Background: Extractions of Lessertia frutescens (Lf)
are shown to have immune modulation, anti-inflammatory and antioxidant
properties. However, Lf is also cytotoxic, antiproliferative, and
pro-apoptotic in vitro. Furthermore, Lf extractions may
influence steroidogenesis. Nevertheless, the impact on Leydig cell function has
not previously been investigated. As tumor necrosis factor-alpha (TNF-
Cytokines influence the development and function of the adrenals, testes, and
ovaries, and further modulate steroidogenesis [1, 2]. Inflammatory cytokines are
elevated in the male reproductive tract during both acute and chronic
inflammatory diseases, contributing to the development of male hypogonadism
[3, 4, 5]. Several cytokines, including the pro-inflammatory cytokine tumor necrosis
factor-alpha (TNF-
Originally discovered as a protein that mediates tumor necrosis with a role in
cancer cachexia [6], TNF-
Several clinically approved protein-based TNF-
Lessertia frutescens (Linnaeus (L.)) Goldblatt and Manning) (abbreviated as
Lf in this manuscript), indigenous to Southern Africa, is traditionally
considered an important medicinal herb [19, 20]. Common local names for
Lf are Sutherlandia or Cancer bush (English), Kankerbos (Afrikaans),
Umnwele (isiXhosa), Insiswa (isiZulu), and Phetola (Sotho) [19]. Numerous
constituents of Lf extractions from leaves have been identified,
including free amino acids (asparagine, proline, and L-arginine), non-protein
amino acids (L-canavanine and
Lf is traditionally used for blood purification (bitter tonic), as an anti-stress remedy, and in the prevention and treatment of cancer [19, 25, 26]. Using in vitro and in vivo experimental models, extractions from Lf have been shown to be hypoglycaemic and antidiabetic [27, 28, 29, 30, 31, 32], immune modulating [31, 33, 34, 35, 36, 37], antioxidant [38, 39, 40], analgesic [31], anti-bacterial [38] and anti-human immunodeficiency virus [41]. Lf has also been classified as an adaptogen, promoting adaptability and resilience to environmental stressors [42] and promoting an anti-stress response [43, 44, 45]. Furthermore, Lf is antiproliferative and pro-apoptotic in cancer cell lines [21, 46, 47, 48, 49, 50, 51, 52, 53].
It is currently unknown what effect Lf has on Leydig cell function. It
is further plausible that Lf may protect against inflammation-mediated
Leydig cell dysfunction. However, no investigation has been done in this
direction. As the impact of Lf on Leydig cell function and dysfunction
remains unclear and poorly investigated, the aim of this study was to investigate
the potential protective effect of Lf extraction on Leydig cells exposed
to TNF-
In this study, an in vitro human chorionic gonadotrophin
(hCG)-stimulated TM3 Leydig cell culture model was used [54]. TM3 cells are an
immortalized Leydig progenitor cell line with epithelial morphology and adherence
culture properties that are derived from 11–13 d mouse testes [55]. The TM3
Leydig cells for laboratory use were purchased without Mycoplasma contamination
from the American Type Culture Collection (Manassas, VA, USA) under the catalog
number CRL-1714 and were cultured according to supplier recommendations as
described below. The cell model was exposed to three different conditions in 25
mIU/mL hCG-enriched cell culture medium for 24 h: (a) increasing concentrations
of TNF-
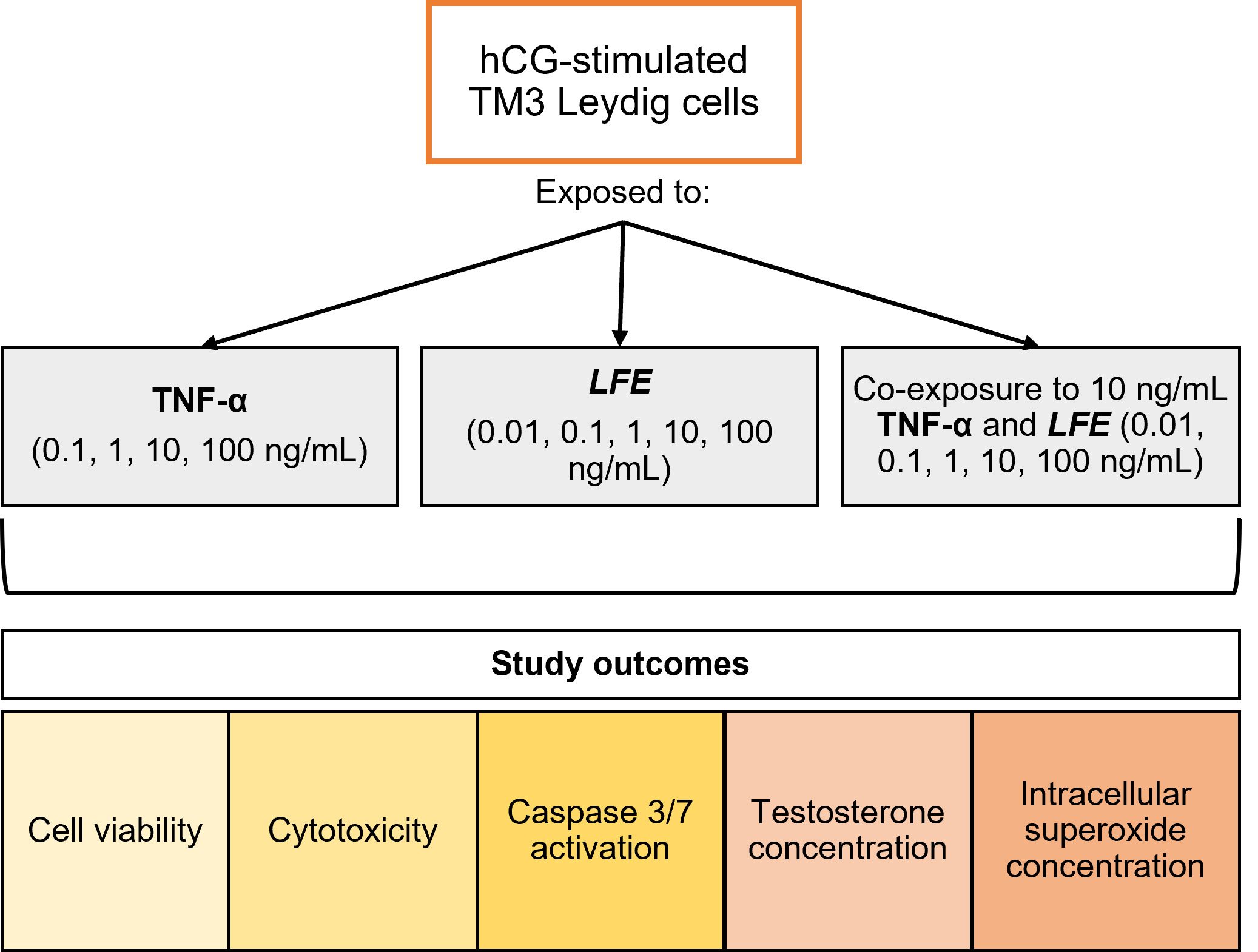
Study design workflow. The figure illustrates the culture
model, experimental conditions, and study outcomes analyzed. LFE,
Lessertia frutescens extract; TNF-
Recombinant TNF-
The plant name has been checked with http://www.theplantlist.org (date of access
December 13, 2022). Lessertia frutescens plants were grown by Parceval
Pharmaceuticals (Wellington, South Africa) as a non-commercial product on the
Ezibusisweni farm without artificial fertilizers, pesticides, or herbicides and
watered 3 times per week. Following harvest, a sample of the fresh plant material
was authenticated as Lf by a botanist, Mr Frans Weitz (Curator of the
Herbarium at the University of the Western Cape, Bellville, South Africa). The
samples were subsequently archived at the Herbarium under the voucher number
UWC6971. Following authentication, the harvested fresh leaves were dried in a
ventilated oven for 3 days (Memmert, Schwabach, Germany) at 35 °C and
milled into finely ground dried leaf material. Extraction of Lf leaves
with hot water has higher total phenolic content, flavonoid content, and total
reducing power (µg/mL dried leaf) compared to cold water, methanol,
ethanol, and acetone [40], with aqueous extractions previously reported
[27, 28, 44, 59]. For this study, Lf underwent extraction
using a glass soxhlet extractor, where 10 g of dried milled leaves were extracted
in 200 mL distilled water (dH
TM3 Leydig cells were cultured using medium that consisted of DMEM/F-12 with
10% fetal bovine serum (Thermo Fisher) and 1% of 50 mg/mL
penicillin-streptomycin (Sigma-Aldrich) [56] in 75 mL flasks. The cells were
incubated in an atmosphere of 5% CO
For each experiment and control, the cell culture medium was supplemented with
25 mIU/mL hCG (Sigma-Aldrich) [54]. Following confluency and detachment, 4.5
Cell viability, cytotoxicity, and caspase 3/7 activation were determined using
the ApoTox-Glo™ Triplex assay (Promega, Madison, WI, USA)
according to the manufacturer’s instructions. The ApoTox-Glo™
Triplex assays were done in white opaque bottom 96-well plates. Following cell
incubation with TNF-
Intracellular superoxide was determined using the dihydroethidium (DHE)
fluorescence stain (Thermo Fisher) in white opaque bottom 96-well plates. After
the termination of the incubation, according to the study design, cells were
washed with phosphate-buffered saline (PBS) (Oxoid, Basingstoke, Hampshire, UK).
Subsequently, the cells were incubated with 10 µM DHE fluorescence
stain in a cell culture medium with 5% CO
The testosterone concentration in the culture medium was determined using the Testosterone ELISA kit (DRG International, Springfield, NJ, USA) according to the manufacturer’s instructions. Following the termination of each experiment, 300 µL of the medium was pipetted into a centrifuge tube and centrifuged at 1500 rpm for 10 minutes to remove excess cells. This supernatant was immediately stored at –20 °C until assayed. In brief, 25 µL of cell supernatant and 200 µL enzyme conjugate were mixed in the antibody-coated microplate and incubated for 1 h at room temperature, along with the standards provided in the kit. The mixture was then removed, and the wells were washed with 400 µL of diluted wash solution 3 times. Subsequently, 200 µL of the substrate solution was added to each well for 5 minutes at room temperature, and the process terminated by adding 100 µL of stop solution. The absorbance was then determined at 450 nm with an ELISA plate reader (Labtech, Heathfield, East Sussex, UK). The final concentration was quantified based on the standard curve determined by the supplied standard concentrations for the assay and represented as ng/mL. Each set of experiments was done with the corresponding control group. As these experiments were done over time, with minor different subsets of cell line passages from frozen storage, absolute values of controls can vary, resulting in a minor variation in the 3 control groups.
In our study, we included 3 biological replicates, which were analyzed in
triplicates for a total of 9 measurements in each experiment. The analysis was
conducted by using MedCalc® statistical software (version 20.109,
Ostend, Belgium). To analyze whether or not the data was normally distributed,
the Shapiro-Wilk test was performed, followed by repeated measures ANOVA or
Friedman test, depending on data distribution. Data was expressed as mean
A decrease in cell viability was observed after exposing the TM3 cells to
increasing concentrations of TNF-
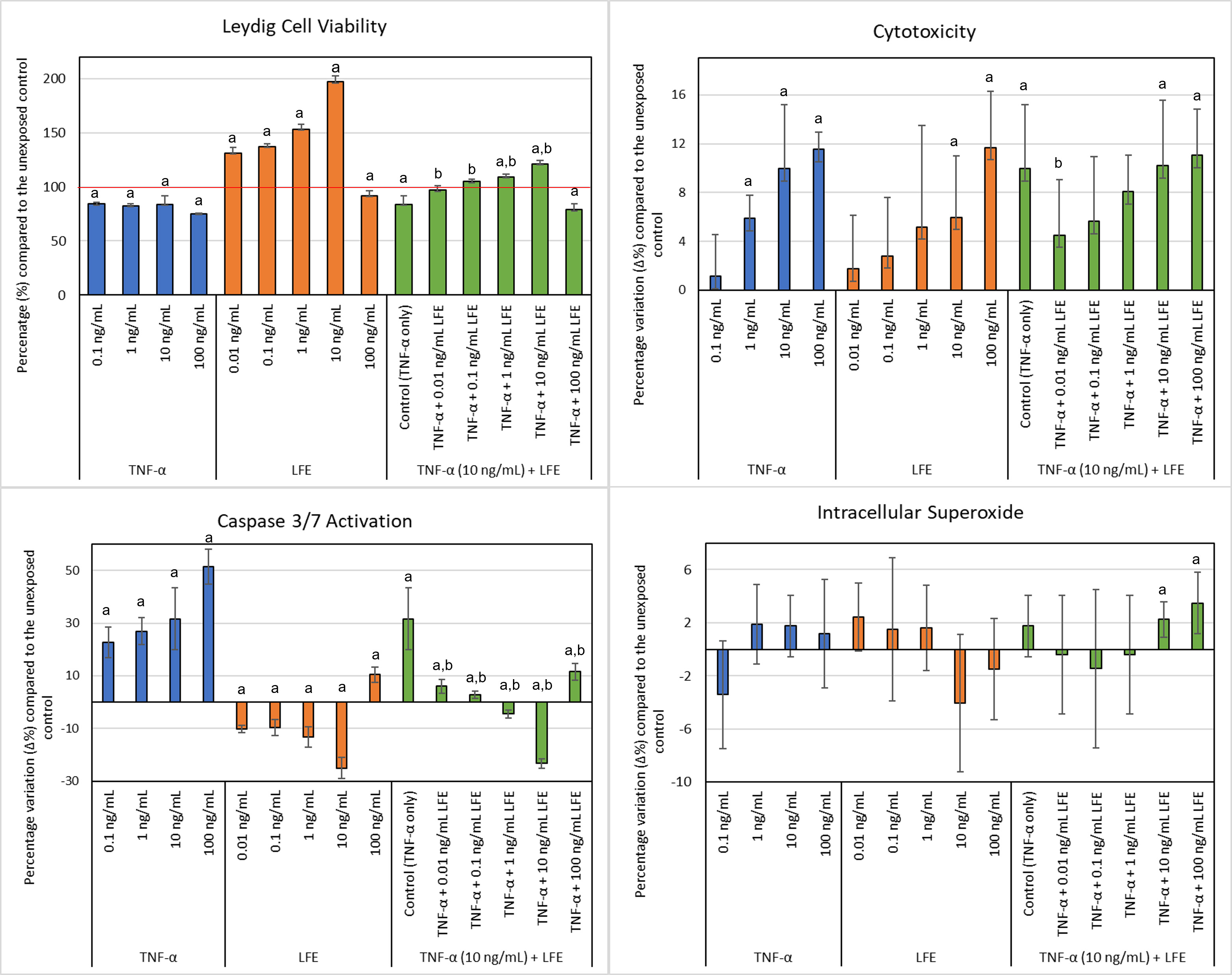
TM3 Leydig cell viability, cytotoxicity levels, caspase 3/7
activation, and intracellular superoxide after exposure to various
concentrations of TNF-
Cytotoxicity did not significantly change with exposure to 0.1 ng/mL
TNF-
Exposure to TNF-
Intracellular superoxide staining did not significantly change after exposure to
TNF-
Testosterone significantly decreased after exposing cells to the lowest
concentration of TNF-
Exposure | Tested groups | Testosterone (Mean |
TNF- |
Control (hCG media only) | 0.29 |
0.1 ng/mL | 0.23 | |
1 ng/mL | 0.25 | |
10 ng/mL | 0.25 | |
100 ng/mL | 0.24 | |
LFE | Control (hCG media only) | 0.24 |
0.01 ng/mL | 0.22 | |
0.1 ng/mL | 0.20 | |
1 ng/mL | 0.22 | |
10 ng/mL | 0.22 | |
100 ng/mL | 0.30 | |
TNF- |
Control (hCG media + TNF- |
0.26 |
TNF- |
0.23 | |
TNF- |
0.26 | |
TNF- |
0.29 | |
TNF- |
0.27 | |
TNF- |
0.39 |
Results are reported as ng/mL. Mean
Since Lf is reported to have modulating effects in vivo and
in vitro on immune function [31, 33, 34, 35, 36, 37], oxidative stress
[38, 39, 40], steroid hormone synthesis [43, 44, 45], and apoptosis
[21, 46, 47, 48, 49, 50, 51, 52, 53], we investigated the effect of aqueous LFE in
protecting Leydig cells against the damage induced by TNF-
The effects of LFE on Leydig cell viability and cytotoxicity have not been previously reported. This study found that LFE increased cell viability at the lower concentrations but decreased viability at the highest one, with a corresponding increase in cytotoxicity. This suggests that the LFE may stimulate Leydig cells at lower concentrations but exerts a cytotoxic effect at higher concentrations used in this study. There are only a few studies on cell viability for LFE in vitro, all of which use higher LFE concentrations compared to this study. SH-SY5Y neuroblastoma cells treated with 20–60 µg Lf for 24 h showed increased cell viability, contrary to reduced viability at a much lower concentration of 100 ng/mL LFE in our study [60]. However, the extraction method is described only as 1 Kg of dried leaves in an unknown volume of boiling water before freeze-drying, and the dosage is provided as an absolute number (20–60 µg) only [60]. In cultured renal LLC-PK1 and MDBK epithelial cells, exposure to an aqueous extraction over 48 h resulted in no significant change in cell viability at concentrations of 0.3–6 mg/mL compared to the reduced viability for LFE at 100 ng/mL, although there was a significant reduction at 12–24 mg/mL [61]. The variation in differences in viability at different dosages across these studies is, however, not clear and may be a response to different cell lines used. This different response of cell lines is further reported, where exposure of normal human lymphocytes to 2.5 mg/mL of ethanolic and aqueous LFE showed an increase in cytotoxicity and decrease in cell viability after 24 h [62], and no effect was observed on LLC-PK1 and MDBK cells at similar dosages [61].
LFE cytotoxicity is more widely reported in cancer cell lines. In prostate cancer in vitro and in vivo, methanolic LFE inhibits cell proliferation [47], similar to what was observed for ethanolic LFE in breast carcinoma and leukemia cells in vitro [21]. MCF-7 breast adenocarcinoma cells have been shown to be more susceptible to cytotoxic effects of 1 mg/mL aqueous LFE (48 h exposure) than non-tumorigenic MCF-12A cells [46]. Similarly, at 1.5 mg/mL, antiproliferative effects and morphological signs of apoptosis were observed in MCF-7 cells after 24 h of exposure [48]. Furthermore, Lf has been shown to cause cytotoxicity in the Salmonella typhimurium strain TA97a, even if this was not evident in strains TA98, TA100, and TA102 [63]. Although our results showed reduced viability and increased toxicity at 100 ng/mL, hot water extracts of Lf leaves did not affect cell viability in Chinese Hamster Ovary (CHO) or Lung Carcinoma Epithelial (A549) cells exposed for 24 h at Lf concentrations up to 500 µg/mL, even if reduced cell viability in HepaRG cells was apparent at concentrations higher than 100 µg/mL [40]. However, it is difficult to compare the results of these different LFE and concentrations on different physiological and pathological cell lines that are currently available in the literature.
Our results suggest that LFE protects cells against TNF-
TNF-
Previously, it has been shown that high concentrations of LFE can be toxic. In cultured renal LLC-PK1 and MDBK epithelial cells, exposure for 48 h to an aqueous LFE significantly increased caspase-3 and -7 activity [61]. In human lymphocytes, 24 h exposure to 2.5 mg/mL of ethanolic and aqueous LFE each induced necrosis, reduced ATP, increased DNA fragmentation, and inhibited caspase 3/7 [62]. However, the apoptotic effect has been more widely reported in cancer cell lines. LFE (2.5 and 5 mg/mL) induced apoptosis in an oesophageal cancer cell line, with increased Annexin V/propidium iodide and caspase-3 and -7 [50]. In SiHa cervix carcinoma cells, a methanol LFE (50 µg/mL–200 µg/mL) reduced cell proliferation and increased caspase 3/7 activity after exposure for 4–24 h [76]. Ethanolic LFE extracts reduced proliferation and induced apoptosis in CaCo2 colon cancer cells, with down-regulation of PI3-K and Akt phosphorylation pathways and decreased forkhead transcription factor (FKHR) phosphorylation [53]. However, this increase in caspase 3/7 with LFE exposure is not always consistent. Ethanolic LFE (0.15–2.5 mg/mL) inducing apoptosis at higher dosages in various melanoma cell lines showed no activation of caspase 3/7, -8, and -9 over a period of 24 h, although this increased after 48 and 72 h of exposure [52]. However, the use of a pan-caspase inhibitor suggested that apoptosis may also occur independently of caspase activation with LFE exposure [52].
In our study, it appears that LFE is protective against TNF-
The apoptotic effect of TNF-
TNF-
There are no previous studies in the literature investigating the effects of
LFE on testosterone synthesis in Leydig cells. However, our results
showed an increase in testosterone at high concentrations of aqueous LFE
(4 mg/mL) orally administered in healthy Wistar rats significantly increased
corticosterone, with no effect on testosterone after 28 days [44]. Studies in
adrenal steroidogenesis, however, suggested mechanisms for modulating
corticosterone and potentially testosterone in these cells. LFE and
sutherlandioside B (10 and 30 µM) inhibited steroidogenic enzymes
and modulated adrenal hormone biosynthesis in COS-1 and adrenal H295R cells [45].
LFE reduced total steroid production, including cortisol, deoxycortisol,
and mineralocorticoid, and inhibited progesterone conversion by CYP17A1 and
cytochrome P450 21
According to our results, LFE may protect against a decline in testosterone at higher concentrations. However, there are very few studies in the literature investigating the effect of LFE on testosterone production. There was no change in testosterone concentration in rats undergoing stress immobilization, although there was an increase in corticosterone in the stressed rats. The administration of aqueous LFE (4 mg/mL) had no significant effect on testosterone concentrations but did reduce corticosterone levels significantly [44]. Although animal studies suggest LFE may inhibit progesterone synthesis from pregnenolone through down-regulated CYP17A1 in adrenal cells [45], the effects on testosterone synthesis in Leydig cells may not be affected. Further investigations into the effect of LFE on testosterone synthesis within the broader context of steroidogenesis in different cell types are required.
Due to the exploratory design of the study, there was a limitation in the number
of variables investigated. Only one concentration of TNF-
This study reports that TNF-
Lf, Lessertia frutescens; LFE, Lessertia frutescens extract;
TNF-
The datasets used and/or analyzed during the current study are available from the corresponding author on reasonable request.
All authors meet the requirements of ICMJE for authorship. KL designed the research study. KL, RH, RF, and CZB performed the research. RF and CZB analyzed the data. KL, RF, and CZB wrote the manuscript. All authors contributed to editorial changes in the manuscript. All authors read and approved the final manuscript.
As an in vitro study, ethical clearance was not required and informed consent is not applicable.
Mr. Lilburn Cyster (Department of Biodiversity and Conservation Biology, University of the Western Cape) to help during the freeze-drying preparation of Lessertia frutescens extract. Dr James Campbell (School of Natural Medicine, University of the Western Cape, Bellville, South Africa) for donation of the Lessertia frutescens obtained from Parceval Pharmaceuticals (Wellington, South Africa) for research purposes. Mr. Frans Weitz (Curator of the Herbarium at the University of the Western Cape) for authentication of Lessertia frutescens.
This study was financially supported by the National Research Foundation (NRF) in Pretoria, South Africa (TTK14051367261).
The authors declare no conflict of interest. The National Research Foundation (NRF) did not influence the collection, analysis, and interpretation of data. Opinions expressed and conclusions arrived at are those of the authors and are not necessarily to be attributed to the NRF.
Publisher’s Note: IMR Press stays neutral with regard to jurisdictional claims in published maps and institutional affiliations.