- Academic Editor
Hemophilia, cancer, diabetes, cardiovascular disease, mental health issues, immunological deficiencies, neuromuscular disease, blindness, and other ailments can all be treated with gene silencing and gene therapy, a growing discipline in medicine. It typically refers to a range of therapeutic techniques in which a patient’s body’s particular cells are given genetic material designed to correct and erase genetic flaws. The advancements in genetics and bioengineering have paved the way for the conceptualization of gene therapy through the manipulation of vectors, enabling the targeted transfer of extrachromosomal material to specific cells. One of the main focus areas of this methodology is the escalation of delivery vehicles (vectors), primarily plasmids or viruses; it still has difficulties because there is no good delivery mechanism that can precisely deliver stable small interfering Ribonucleic Acid (siRNA) or DNA to the target tissue location. As they are non-fluorescent, the siRNA or DNA delivery procedure is unable to be monitored by these carriers. In the context of quantum dots (QDs), the formation of QD-siRNA or QD/DNA complexes facilitated the real-time monitoring and precise localization of QDs during the silencing, delivery, and transfection processes. The unique dual-modality optical and fluorescent properties exhibited by quantum dots contribute to their utility as versatile imaging probes. The research studies discussed in this review article will provide a framework for designing efficient QD-based nanocarriers that can successfully carry therapeutic genetic tools into targeted cells. As a result of their findings, the researchers developed some unique QDs that successfully attached to the siRNA or DNA and carried it to the desired place. The use of these QD-based delivery devices could enhance the field of gene silencing and gene delivery.
Quantum dots (QDs) are nano-sized semiconductor crystals, typically ranging from 1 to 10 nanometers in diameter, and they exhibit exceptional optical properties that are central to this burgeoning scientific progress and an arising topic in the field of nanotechnology imparting a great effect on science by collaborating with biology. They were initially discovered in the 1980s by physicist Alexei Ekimov, who devoted his research to the study of semiconductors [1]. The classification of QDs into distinct categories is primarily determined by their chemical composition and the arrangement of constituent elements within the periodic table. Depending on these factors, QDs can be categorized into 12 different classes, each exhibiting unique properties and characteristics. For instance, QDs belonging to group IV A elements encompass carbon (C), silicon (Si), and germanium (Ge). These elements are tetravalent, with four electrons in their outermost electron shell. Indeed, the elements C, Si, and Ge share common physicochemical characteristics due to their metalloid nature and semiconducting electrical properties [2]. The majority of QDs used in various applications exhibit a specific chemical composition characterized by a core-shell structure. The core consists of a heavy metal element, such as lead selenide (PbSe), cadmium telluride (CdTe), zinc selenide (ZnSe), or cadmium sulfide (CdS). To enhance stability, biocompatibility, and fluorescence efficiency, a bandgap semiconductor shell is enveloped around the heavy metal core. This semiconductor shell, often composed of silicon dioxide (SiO2), serves as a protective barrier, shielding the heavy metal core from direct exposure to the surroundings [3, 4].
Undeniably, within the realm of Quantum Dots (QDs), there are two notable
exceptions based on a single semiconductor element, such as Silicon Quantum Dots
(Si QDs) and QDs made from semiconducting polymers, also known as P dots. The
general characteristics of QDs, which include their small particle size, high
quantum yield, tunable composition and properties, intermittent light emission
(blinking), and high brightness, have attracted them across an array of
applications, including LED technology, solar cells, and biomedical ones like
drug delivery, imaging, and cancer photodynamic therapy [5, 6, 7]. Some of the
commercialized goods based on QDs technology for biosensor medical applications
have been out in the global market, such as NANOCO
QDs exhibit distinctive electronic behavior in contrast to bulk semiconductors, primarily owing to their extremely small size, which can be on the order of nanometers. This nanoscale dimensionality imparts unique quantum mechanical properties to QDs, akin to those found in single atoms, setting them apart from conventional bulk semiconductors. The tiny size resembling atoms in QDs gives rise to a fascinating phenomenon called “quantum confinement”, in which the band gap energy—i.e., a comparatively substantial amount of energy is required to excite one electron from the low-energy valence band to the high-energy conduction band. The band gap energy in Quantum Dots (QDs) increases for smaller QDs and decreases for larger QDs. This leads to a rise in the energy of the emitted photon, resulting in a shorter emission wavelength. Consequently, the optical behavior of QDs is primarily determined by their size rather than the material they are made of. This size-dependent property enables precise control over the electromagnetic radiation of QDs across a broad spectrum, from ultraviolet (UV) to far infrared, simply by adjusting the particle size [11, 12]. Quantum Dots (QDs) demonstrate a strong “Stokes shift” effect, where there is a noticeable separation between the excitation and emission spectra. When exciting QDs, energy is absorbed at a shorter wavelength, leading to the promotion of electrons. In contrast, the emitted light occurs at a longer wavelength. This Stokes shift phenomenon offers several advantages in practical applications. Firstly, it improves detection sensitivity by reducing interference from scattered excitation light during fluorescence measurements. Secondly, it decreases optical overlap between the excitation and emission spectra, preventing cross-talk and enhancing the accuracy and specificity of fluorescence-based measurements. The Stokes shift effect enhances QDs’ effectiveness as fluorescence probes in various scientific and biomedical applications [13, 14].
The methods for producing quantum dots are divided into two categories: “top-down” (Electrochemical oxidation, Laser ablation, Ultrasonic synthesis, Chemical oxidation) and “bottom-up” (Thermal decomposition, Microwave synthesis — Scheme 1, Templated method, Hydrothermal treatment) [15]. Quantum dots are frequently used for various biomedical-oriented fields, particularly in bioimaging, diagnostics, drug delivery, single molecule probes, and drug analysis, including drug targeting and enhancing drug bioavailability [16], among many other areas, according to recent research or data-based data [17]. However, cutting-edge medicine and biomedical research have undeniably raised people’s quality of life. The treatment with nanodots in cancer, immune disorders, neurological conditions, more importantly, gene therapy helps significantly rather than with the conventional diagnosis and treatment of such illnesses [18]. Gene therapy normally states a group of therapeutic methods in which a patient’s body’s specific cells receive a genetic material that is intended to repair and eradicate genetic defects [19, 20]. Gene therapy has evolved into a promising treatment approach for numerous human diseases nearly five decades after the concept was initially proposed. The road to success has been arduous. Early clinical studies experienced severe adverse effects, but this fueled basic research that resulted in safer and more effective gene transfer vectors. Patients with a range of inherited and acquired illnesses, such as diabetes, cancer, hemophilia, cardiovascular conditions, immune deficiencies, mental disorders, neuromuscular disease, blindness, and other diseases, have benefited clinically from various forms of gene therapy. The utilization of nucleic acids as gene therapy modalities, for example, mRNA, plasmid DNA (pDNA), and noncoding RNAs, is quickly making its way into clinical practice [21].
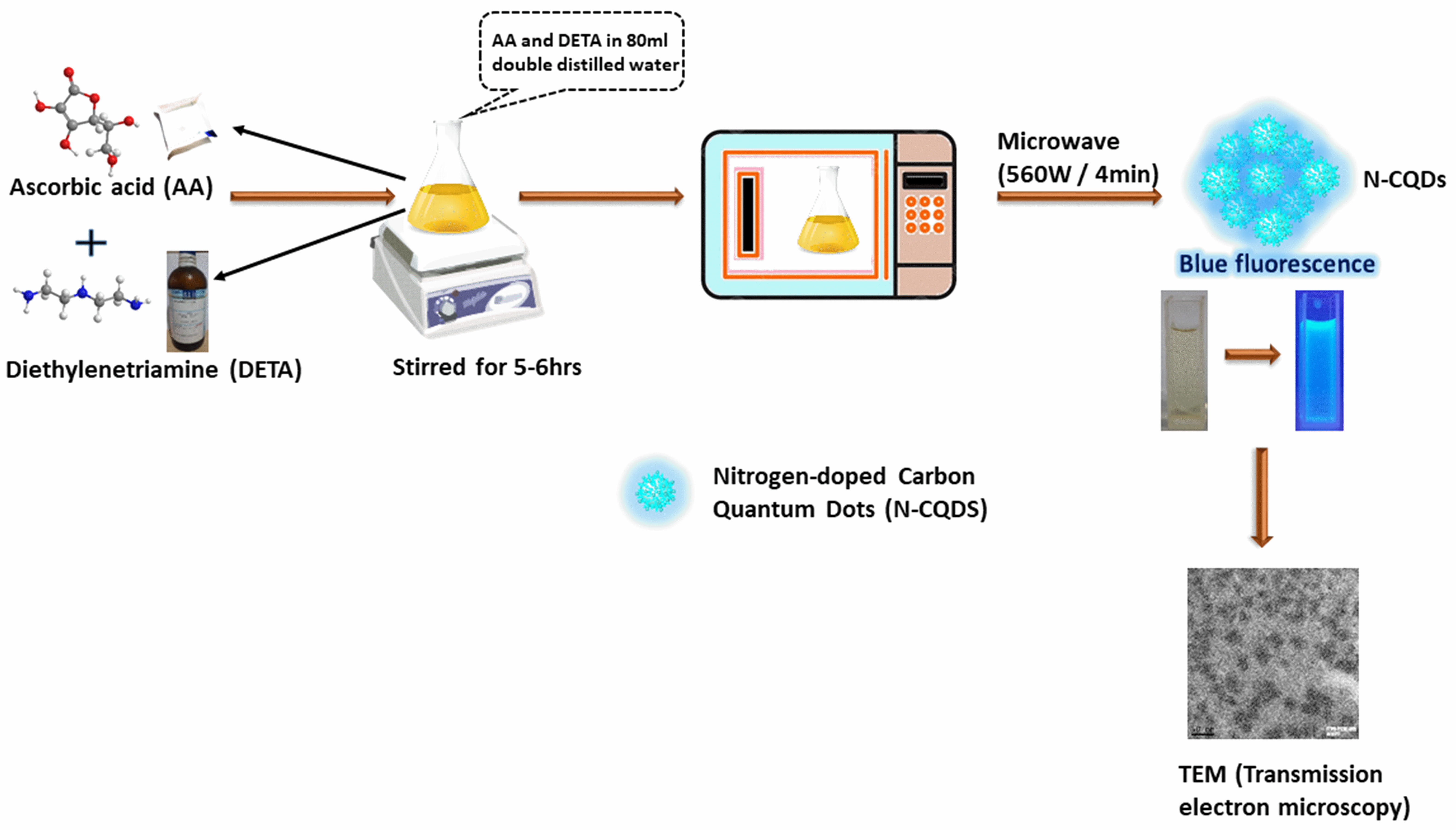
Schematic representation of producing Quantum Dots through the microwave process and its microscopic images.
In this review, we summarized different types of quantum dots used in gene delivery and gene silencing. Over the past few decades, this discipline has made excellent progress, and numerous obstacles have been surmounted in the quest to create novel, effective, and non-toxic quantum dots for gene therapy. Here, we have explored various distinctive approaches to designing different types of quantum dots and their potential applications in gene therapy and gene silencing. As far as we are aware, no recent review articles have explored such unique strategies collectively.
Gene therapy was first discovered in the 1980s, a few years after genes were first isolated from DNA. In 1970, the discovery of certain enzymes allowed for the precise separation and repeatable reinsertion of genes at specific locations along the DNA molecule. These breakthroughs in genetic manipulation laid the foundation for genetic engineering, opening up avenues for the creation of new medications, antibodies, and the potential for gene therapy [22].
The most straightforward definition of gene therapy is the genetic alteration of cells to achieve a therapeutic outcome. Ex-vivo approaches (carrying out such genetic alterations in cultured cells, which are then administered to the patient for therapeutic purposes) or In-vivo approaches (carrying out such alterations in living cells) are both possible. In the majority of early studies of gene therapy, patients with single-gene genetic disorders had their defective gene attempted to be replaced with a functional copy of the gene [23]. Gene therapy entails the deliberate incorporation of a normal and functional gene into the genome of affected cells to substitute or rectify an aberrant gene accountable for a specific disease. One of the major challenges in the process is effectively delivering the gene into the stem cell. For successful gene delivery, a “vector”, which is a molecular carrier, must possess specific attributes. It should exhibit high specificity in releasing one or more genes of sizes suitable for clinical applications. Additionally, the vector must evade immune recognition and be purified in large quantities and high concentrations to enable large-scale production and accessibility (Fig. 1). After the vector has been introduced, it mustn’t induce allergic reactions or inflammatory responses. Rather, the vector should promote beneficial functions, compensate for deficiencies, or inhibit harmful behaviors within the target cells. This ensures that the gene therapy approach is safe and effective in achieving the desired therapeutic outcomes. Moreover, the vector must demonstrate safety for both the patient and the environment, as well as for the healthcare professionals administering the therapy. Additionally, the vector should be capable of sustained gene expression throughout the patient’s lifetime, ensuring long-term therapeutic benefits and minimizing the need for repeated treatments [22, 24].
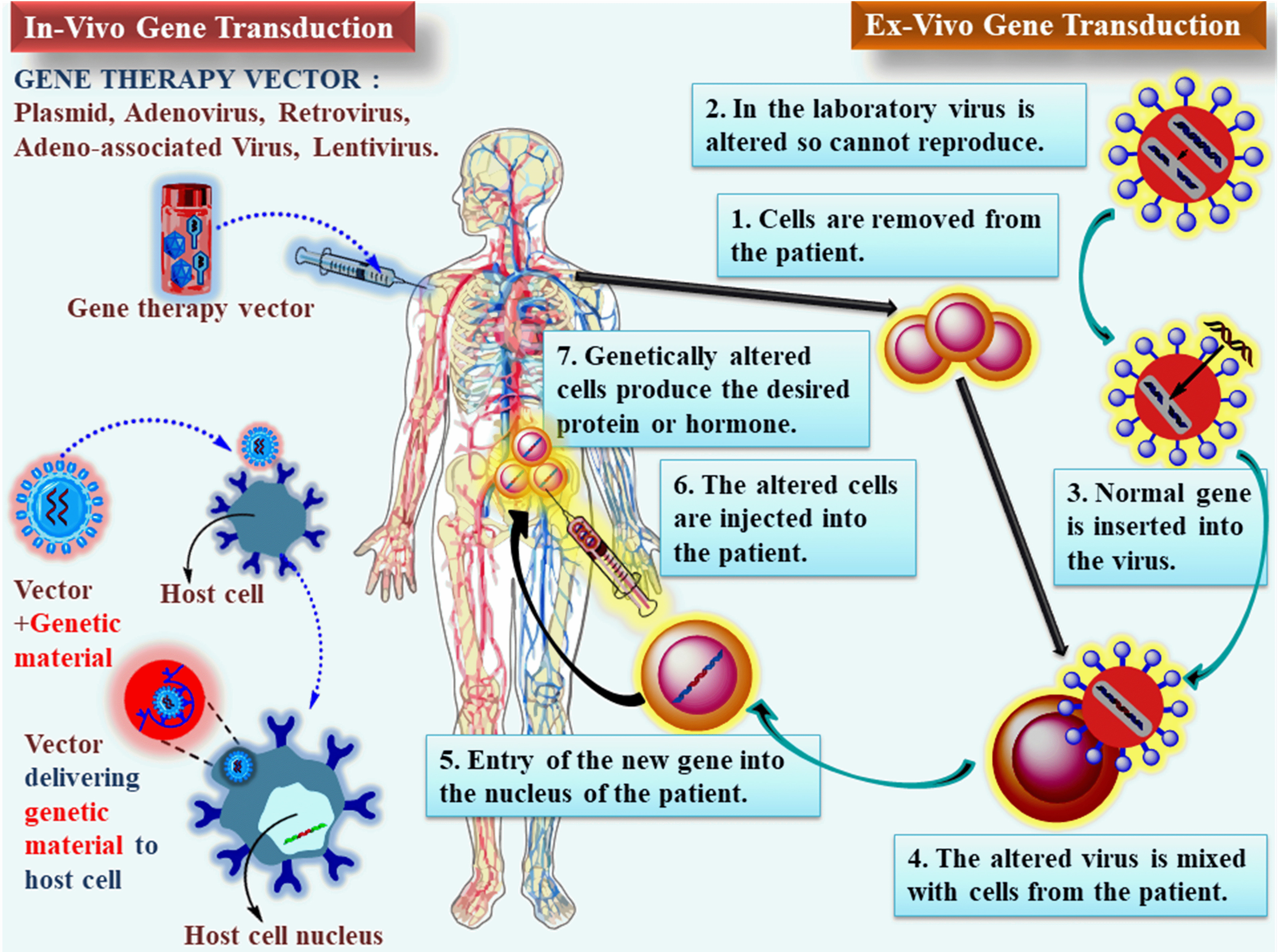
Approach to gene therapy using Ex-vivo and In-vivo: A device is employed to transport the gene to the target organ once it has been encapsulated within one of several vectors. The gene is inserted into a plasmid and subsequently administered to the patient (on the left). Ex-vivo methods involve removing cells from the target tissue, genetically modifying them in a lab dish, and after genetically modifying the cells, they are re-administered to the patient. The vectors utilized for In-vivo gene transduction can also facilitate gene transduction In-vitro.
RNA interference (RNAi) therapy still faces difficulties owing to the absence of a reliable delivery system capable of effectively delivering stable small interfering RNA (siRNA) In-vivo and exhibiting high selectivity for the target tissue region. The methods used today to transport siRNA include liposomes, virus-based vectors, polymers, peptides, etc. Nonetheless, these carriers do not possess the capability to track the siRNA delivery process due to their non-fluorescent nature. Regular methods for measuring siRNA distribution involve co-transfecting reporter plasmids or monitoring fluorescently end-modified siRNA. Nevertheless, these techniques faced issues such as rapid photobleaching, inability to monitor multiple siRNA molecules simultaneously, and lack of sensitivity to various heterogeneous siRNA delivery methods. Quantum dots exhibit distinct optical characteristics that set them apart, including exceptional resistance to photobleaching, the ability to tune their fluorescent peaks based on size, and a broad excitation spectrum coupled with a narrow emission spectrum; these are recently being employed as co-transfection reagents or delivery systems for tracking the distribution of siRNA. The distinctive optical properties of quantum dots make them well-suited for in situ real-time monitoring of the siRNA delivery process [25]. The advantages and disadvantages of mostly used viral and non-viral vectors for gene delivery are summarized in Table 1.
Vector | Advantages | Disadvantages |
Viral | ||
Retrovirus | ||
Adenovirus | ||
Lentivirus | ||
Adeno-associated virus | ||
Non-Viral | ||
Gold nanoparticles | ||
Quantum Dots | ||
Liposome | ||
Poly( |
||
The employment of diagnostic tools and therapeutic modalities in the treatment of human diseases like cancer is typically sequential. This paradigm may shift as a result of multifunctional platforms that combine therapeutic and diagnostic imaging functions into a single machine (Scheme 2). Particularly, multifunctional nanoparticle-based systems can enhance drug formulation pharmacokinetics and enable the incorporation of disease-targeting and diagnostic imaging characteristics. They used these ideas to administer small interfering RNA (siRNA) treatments in situations where systemic delivery is impeded through quick excretion and non-targeted tissue distribution.
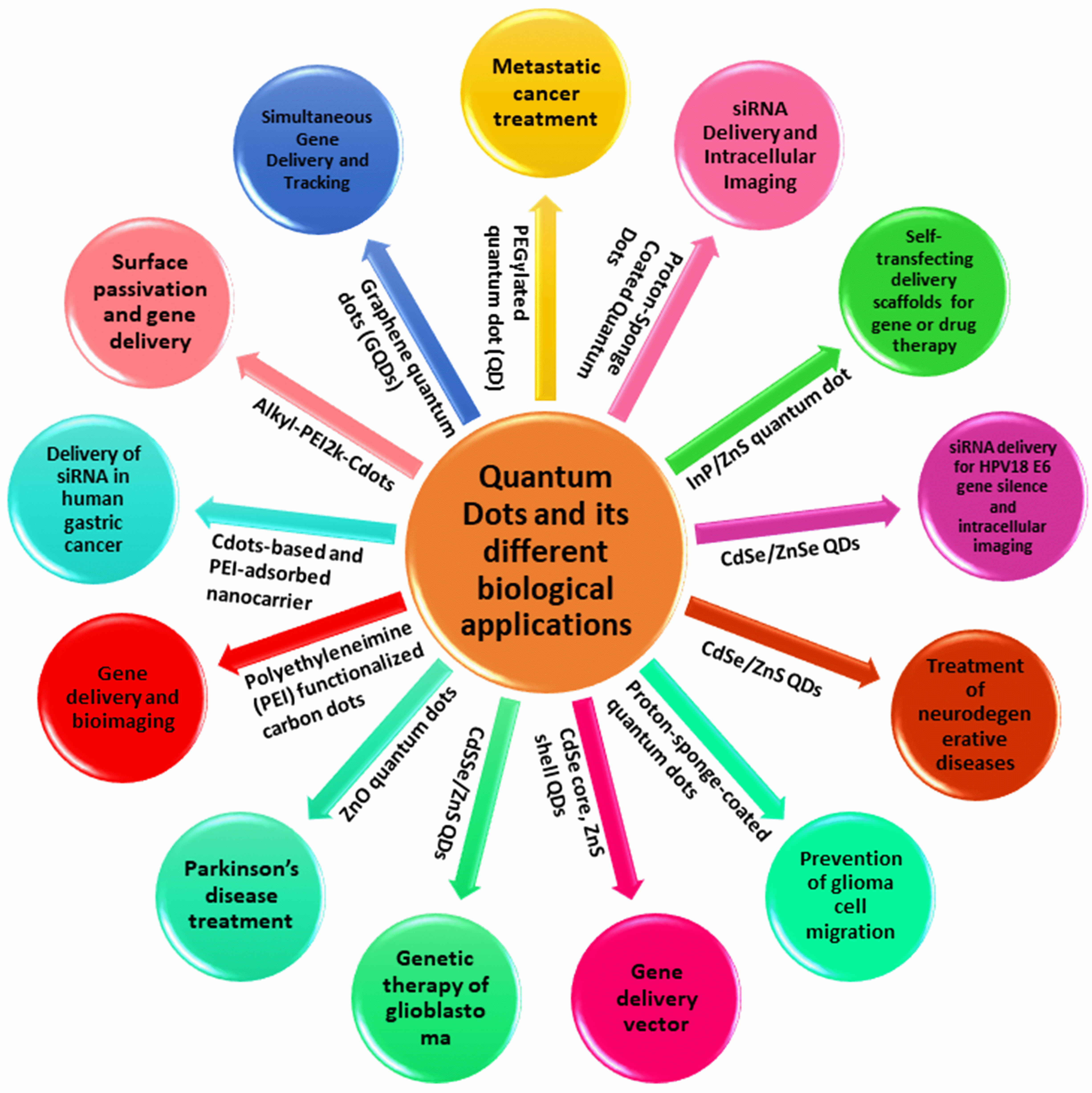
The uses of different quantum dots and their different biological applications.
In 2007, Derfus et al. [26] utilized a PEGylated quantum dot (QD) core as a scaffold, to which they attached siRNA and tumor-homing peptides (F3) through functional groups on the particle’s surface (Fig. 2). Their discoveries revealed the crucial role of the homing peptide in aiding the targeted internalization of siRNA cargo into tumor cells. Furthermore, they observed that co-attaching siRNA cargo did not impede the peptide’s ability to carry out its intended function. The impact of conjugation chemistry was investigated by employing an enhanced green fluorescent protein (EGFP) model system, where siRNA was attached to the particle using disulfide (sulfo-LC-SPDP) and covalent (sulfo-SMCC) cross-linkers demonstrating higher silencing efficiency compared to attachment via a nonreducible thioether linkage (Fig. 3). Further, they conducted a detailed investigation into the interplay between the quantity of F3 peptides and siRNA on each particle. As a result, they achieved an optimized formulation considering that each particle can accommodate only a limited number of attachment sites for the peptides and siRNA. EGFP signal was significantly knocked down upon delivering F3/siRNA-QDs to EGFP-transfected HeLa cells and subsequently releasing them from endosomal entrapment. In the future, this technology has the potential to be adapted for both treatment and imaging of diseases like cancer. By customizing the siRNA sequence to target specific therapeutic goals, such as oncogenes, instead of EGFP, it could be harnessed for precise and targeted therapy along with imaging capabilities across diverse disease conditions [26].
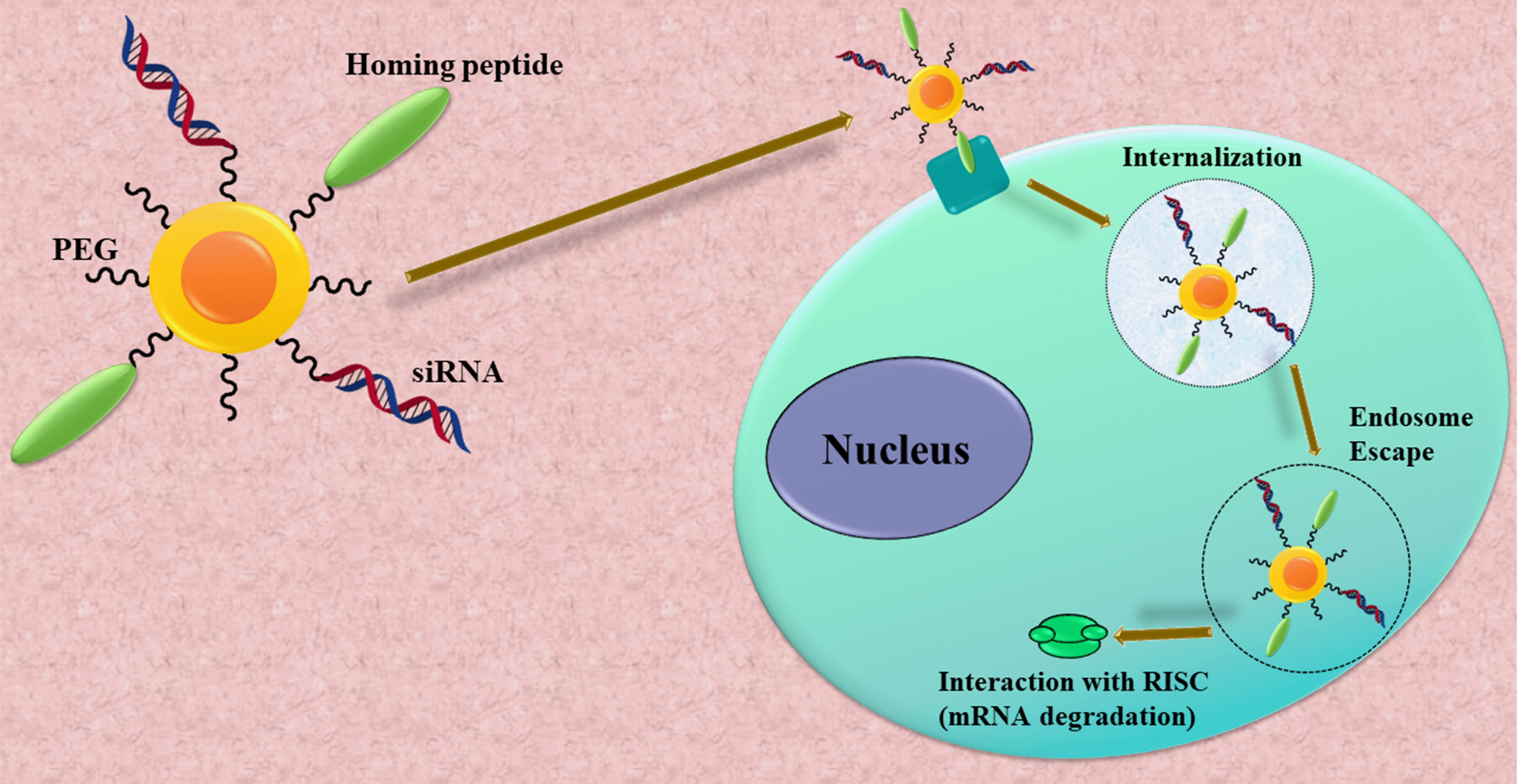
A multifunctional nanoparticle was created to facilitate small interfering Ribonucleic Acid (siRNA) delivery. Quantum dots (QDs) were identified as suitable carriers for transporting siRNA into living cells both In-vitro and In-vivo due to their photostable fluorescence and multivalency. The conjugation of homing peptides to the QD surface (together with the siRNA cargo) enables targeted internalization in tumor cells. After cellular uptake, these particles must evade the endolysosomal route and enter the cytoplasm. In the cytoplasm, they interact with the RNA-induced silencing complex (RISC), which leads to the degradation of messenger Ribonucleic Acid (mRNA) homologous to the siRNA sequence. PEG, polyethylene glycol.
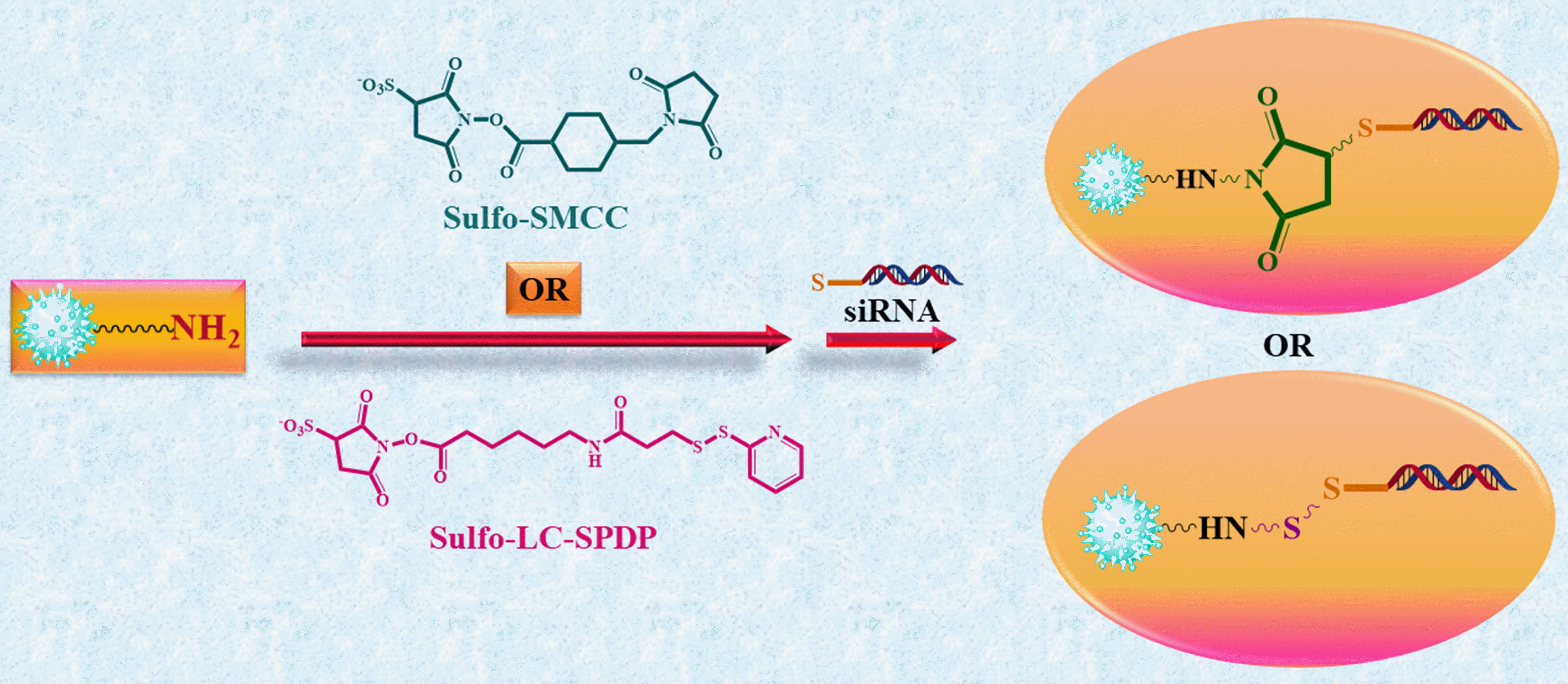
Cross-linking siRNA to QDs using cleavable or non-cleavable
cross-linkers. The water-soluble heterobifunctional cross-linkers sulfo-SMCC
(sulfosuccinimidyl 4-(N-maleimidomethyl)cyclohexane-1-carboxylate) and
sulfo-LC-SPDP (sulfosuccinimidyl
6-(3
In 2008, Yezhelyev et al. [27] presented a methodical approach for developing multifunctional nanoparticles consisting of semiconductor quantum dots (QDs) and proton-absorbing polymeric coatings (proton sponges) measuring 6 nm in size, as observed through Transmission Electron Microscopy (TEM). These nanoparticles were designed to lubricate the short-interfering RNA (siRNA) delivery and imaging. The nanoparticles were meticulously designed with a well-balanced composition of tertiary amine and carboxylic acid groups to overcome longstanding barriers in siRNA delivery, such as endosomal release, intracellular transport, cellular penetration, and carrier unpacking (Fig. 4). The hydrodynamic diameters of the proton-sponge dots are approximately 13 nm before siRNA binding, and they increase to about 17 nm after siRNA binding. When compared to existing transfection agents for MDA-MB-231 cells, the results indicate a remarkable 10–20-fold improvement in gene silencing efficiency, with a substantial 5–6-fold reduction in cellular toxicity. To assess cellular toxicity, the Sulforhodamine B (SRB) assay was employed to compare proton-sponge-coated QDs with commercial transfection agents. The results revealed that the proton-sponge-coated QDs exhibited almost negligible toxicity to MDA-MB-231 cells. In addition, the QD-siRNA nanoparticles act as probes with dual-modality optical and electron-microscopy functionality, enabling the precise localization of QDs within their ultrastructure and real-time tracking of their movement during delivery and transfection processes. These novel findings and capabilities imply significant progress in the realm of nanoparticle engineering, propelling their potential for imaging and therapeutic applications [27].
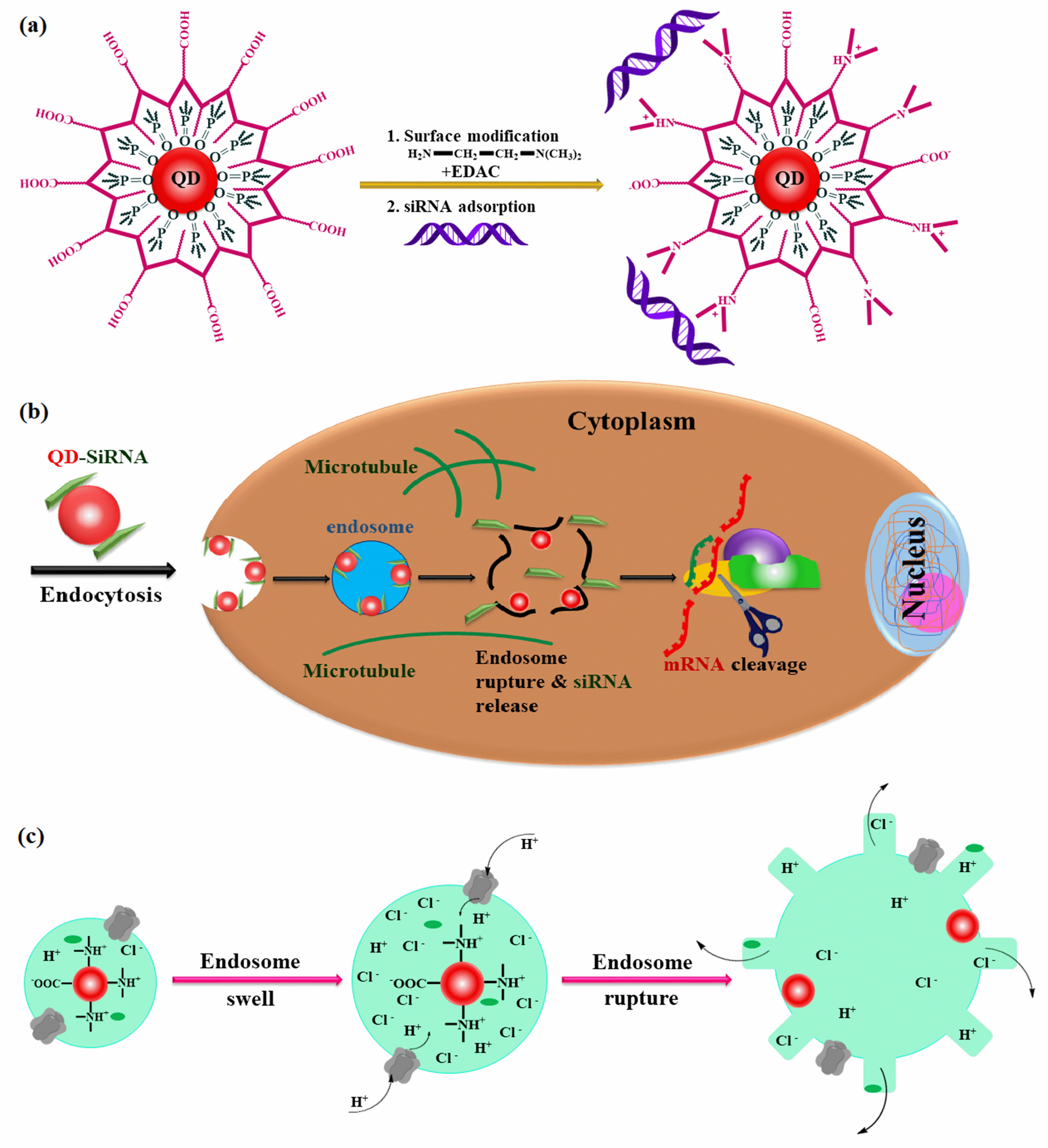
Proton-sponge coated quantum dots: rational design and application as a multifunctional nanoscale carrier for siRNA administration and intracellular imaging. (a) Chemical alteration of polymer-encapsulated QDs to introduce tertiary amine groups, and electrostatic adsorption of siRNA on the particle surface. (b) Schematic depiction of siRNA-QD membrane binding, cellular entry, endosomal escape, capture by RNA binding proteins, loading into RNA-induced silencing complexes (RISC), and target destruction. (c) Diagram of the proton-sponge action, demonstrating the participation of the membrane protein ATPase (proton pump), osmotic pressure buildup, and organelle swelling and rupture.
Specially designed functional quantum dots (QDs) were developed to overcome
siRNA delivery challenges, including cellular penetration, intracellular
transport, carrier unpacking, endosomal release, siRNA protection, and gene
silencing. In 2011, Li et al. [28] developed a new category of
multifunctional complexes for siRNA delivery and intracellular imaging. These
complexes were created by employing direct ligand-exchange reactions to load
CdSe/ZnSe QDs onto L-Arg (L-arginine) and
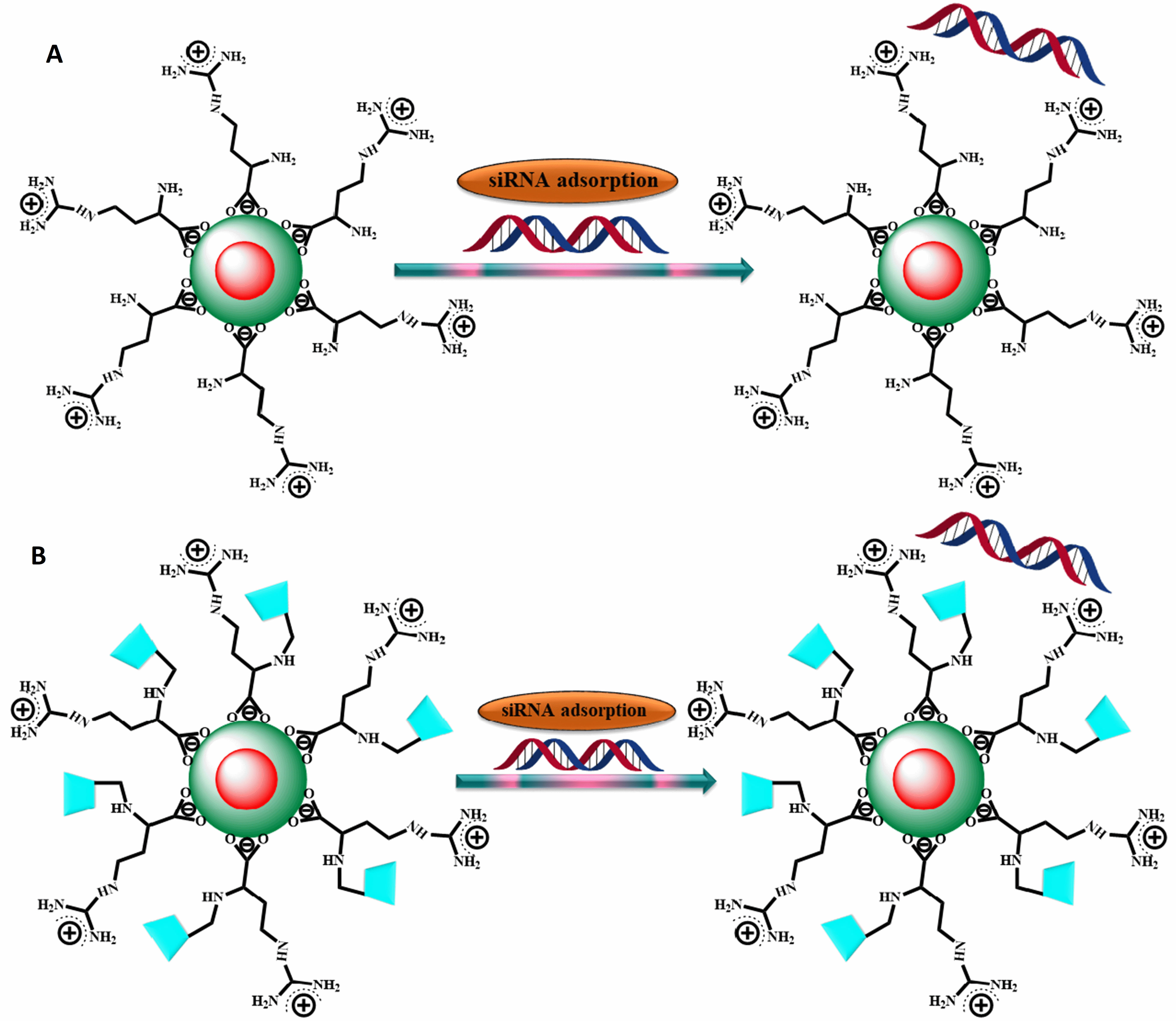
Adsorption of siRNA onto L-arginine-functional. QDs (A) and
L-arginine-functional
The main driver of Alzheimer’s disease (AD) pathogenesis is the accumulation of
In 2012, Li et al. [39] developed a Quantum-siRNA nanoplex to silence
the BACE1 gene and perform intracellular imaging in SK-N-SH Cells (human
neuroblastoma cell line). Modified CdSe/ZnS QDs were synthesized by introducing
amino-polyethylene glycol (PEG-QDs), and subsequently, QD-PEG/siRNA nanoplexes
were formed by electrostatically adsorbing negatively charged siRNAs onto the
surface of QDs (Fig. 6). TEM image revealed that the QDs were monodisperse with a
diameter of 5
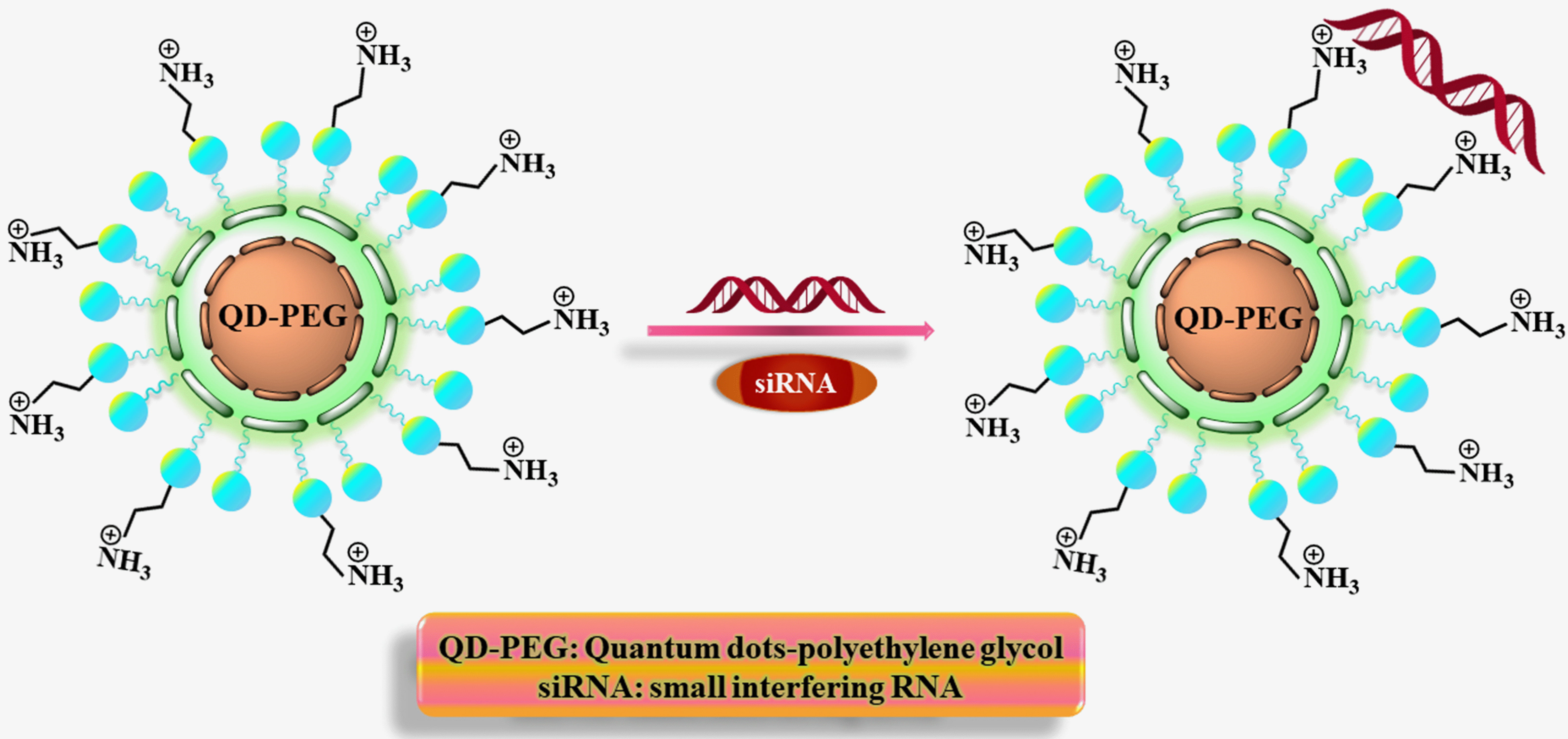
The formation of QD-PEG/siRNA complexes is depicted diagrammatically.
In 2013, Amphipol PMAL, which serves as an encapsulating polymer, was grafted with polyethyleneimine (PEI) to create proton-sponge coated quantum dots by Qi et al. [25] (Fig. 7). TEM imaging reveals that the initial QDs exhibit uniform dispersion and possess a core size of approximately 10 nm, and this size remains unchanged in QD-PMAL and QD-PMALPEI configurations. Dynamic light scattering (DLS) measurements indicated that the original QDs had a hydrodynamic diameter of about 12.1 nm, while QD-PMAL and QD-PMAL-PEI had hydrodynamic diameters of approximately 14.7 nm and 32.5 nm, respectively. A standard MTT experiment was carried out to assess the cytotoxicity of the QD-PMAL-PEI and QD-siRNA complexes. In serum-containing media, the QD-PMAL-PEI nanoparticles demonstrated negligible toxicity and remarkable efficiency in silencing the junctional adhesion molecule-2 (JAM-2) gene, which is prominently found in glioma cells. Confocal microscopy examination revealed that siRNA was efficiently released intracellularly. For the first time, it is noted that QD-mediated JAM-2 knockdowns facilitated the prevention of glioma cell migration. Moreover, downregulation of the JAM-2 gene’s downstream genes HES1 and HES5 indicated that the Notch pathway was the JAM-2 gene’s intended target. Besides their role as multifaceted carriers for siRNA, enabling efficient gene silencing and real-time intracellular imaging, the unique proton-sponge coated QDs offer promising potential for the advancement of novel and biocompatible siRNA delivery systems [25].
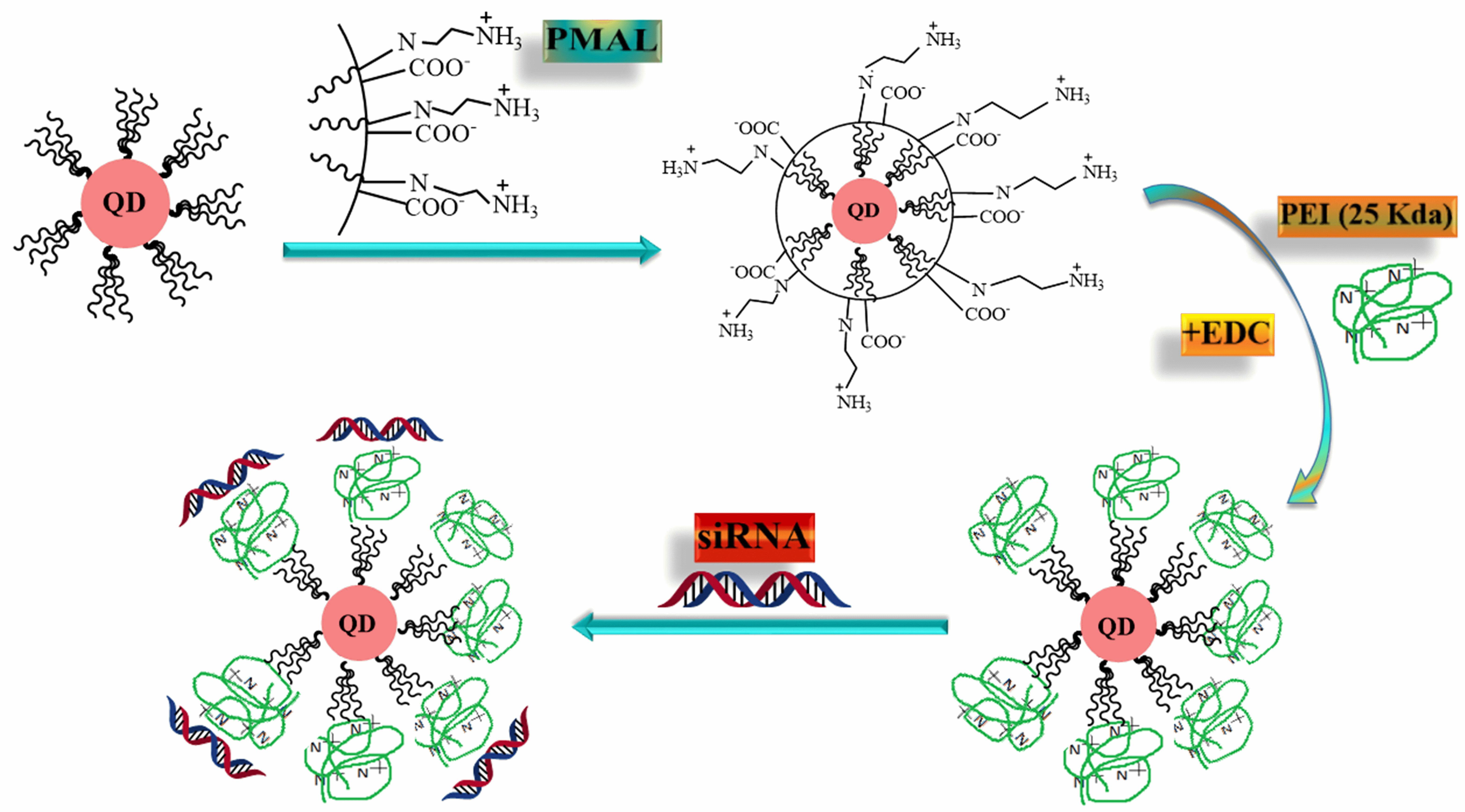
QD-PMAL-PEI synthesized for siRNA delivery illustrated schematically. PMAL, polymaleic anhydride-alt-1-decene; PEI, polyethyleneimine.
The occurrence and growth of human tumors are caused by genetic abnormalities, which then cause apoptosis. Survivin is a recent addition to the family of proteins that suppress apoptosis, inhibit caspases, and prevent cellular apoptosis. The majority of normal, non-diverging adult tissues have very modest levels of survivin expression to significantly and preferentially upregulated cancer cells. The biochemical processes of apoptosis and proliferation are programmable; studies have shown that introducing survivin short interfering RNA (siRNA) into cells can effectively suppress survivin production, which consequently causes a huge rise in the rate of apoptosis. Survivin presents a promising therapeutic target to suppress cancer cell development due to its distinct expression in cancer cells in contrast to normal tissue and its essential function in regulating cancer cell division (proliferation) and survival.
In 2013, Zhao et al. [40] conducted research to investigate the potential of quantum dots (QDs) as non-viral gene vectors for delivering survivin siRNA and downregulating survivin gene expression in oral squamous cell carcinoma Tca8113 cells. In their study, the researchers employed water-dispersible cationically modified QDs to electrostatically bind to anionic siRNA molecules to reduce the expression of the survivin gene. The cellular uptake and distribution of QD-siRNA complexes in Tca8113 cells were observed through confocal laser scanning microscopy. Real-time polymerase chain reaction (PCR) was utilized to quantify the levels of survivin messenger RNA (mRNA). High-intensity fluorescence was used to view CdSe QDs while utilizing confocal laser scanning microscopy. Six hours after the incubation, Tca8113 cells effectively transfected with CdSe QDs carrying survivin siRNA exhibited clear red fluorescence from the QDs and green fluorescein amidite fluorescence from the siRNA. Reduced survivin mRNA levels detected by real-time PCR quantification supported the deliverance of siRNA into the cytoplasm. In this study, survivin siRNA effectively suppressed the expression of the survivin gene in Tca8113 oral squamous cell carcinoma cells and formed stable complexes with water-soluble CdSe QDs, which exhibited strong fluorescence characteristics. This demonstrates the potential of CdSe QDs as a distinctive non-viral vector for gene delivery [40].
On the other hand, a highly promising approach for cancer gene therapy involves RNA interference (RNAi) through the use of short interfering RNA (siRNA). Nevertheless, the clinical application of RNAi remains limited due to the scarcity of effective and safe carriers for delivering siRNA. The most fatal CNS (central nervous system) tumor is glioblastoma because of its aggressiveness. Through the implementation of gene therapy, the siRNA-based RNAi technology shows promising potential in providing patients with a means to combat this deadly disease. The medical research community has made great efforts to find efficient gene targets for the treatment of glioblastoma, and many approaches have been suggested and identified. As telomerase is highly prevalent in the majority of tumor cells, anti-tumor therapeutics targeting telomerase reverse transcriptase (TERT) are used in Guimiao Lin’s study as an innovative therapeutic strategy.
In 2017, Guimiao Lin et al. [41] created nanocarriers for
In-vitro gene delivery based on cadmium sulphoselenide/zinc sulfide
quantum dots (CdSSe/ZnS QDs). When functionalized with polyethyleneimine (PEI),
these CdSSe/ZnS QDs create a stable nanoplex (QD-PEI) capable of loading siRNA
and specifically targeting human telomerase reverse transcriptase (TERT). DLS
results showed that QDs have a hydrodynamic diameter of 12.97
In 2014, Wang et al. [42] developed a nanocarrier called Cdots@PEI by
adsorbing polyethyleneimine (PEI) onto carbon dots (C-dots). This nanocarrier was
designed for the delivery of small interfering RNA (siRNA) against the
antiapoptotic protein Survivin in the human gastric cancer cell line MGC-803
(Fig. 8). The Cdots were developed through a microwave-assisted process, where
citric acid served as the carbon source, and tryptophan acted as both the
passivation agent and nitrogen source. The resulting Cdots exhibited water
dispersibility, biocompatibility, and a high quantum yield. The siRNACdots@ PEI
complexes had hydrodynamic particle sizes that were 4.7
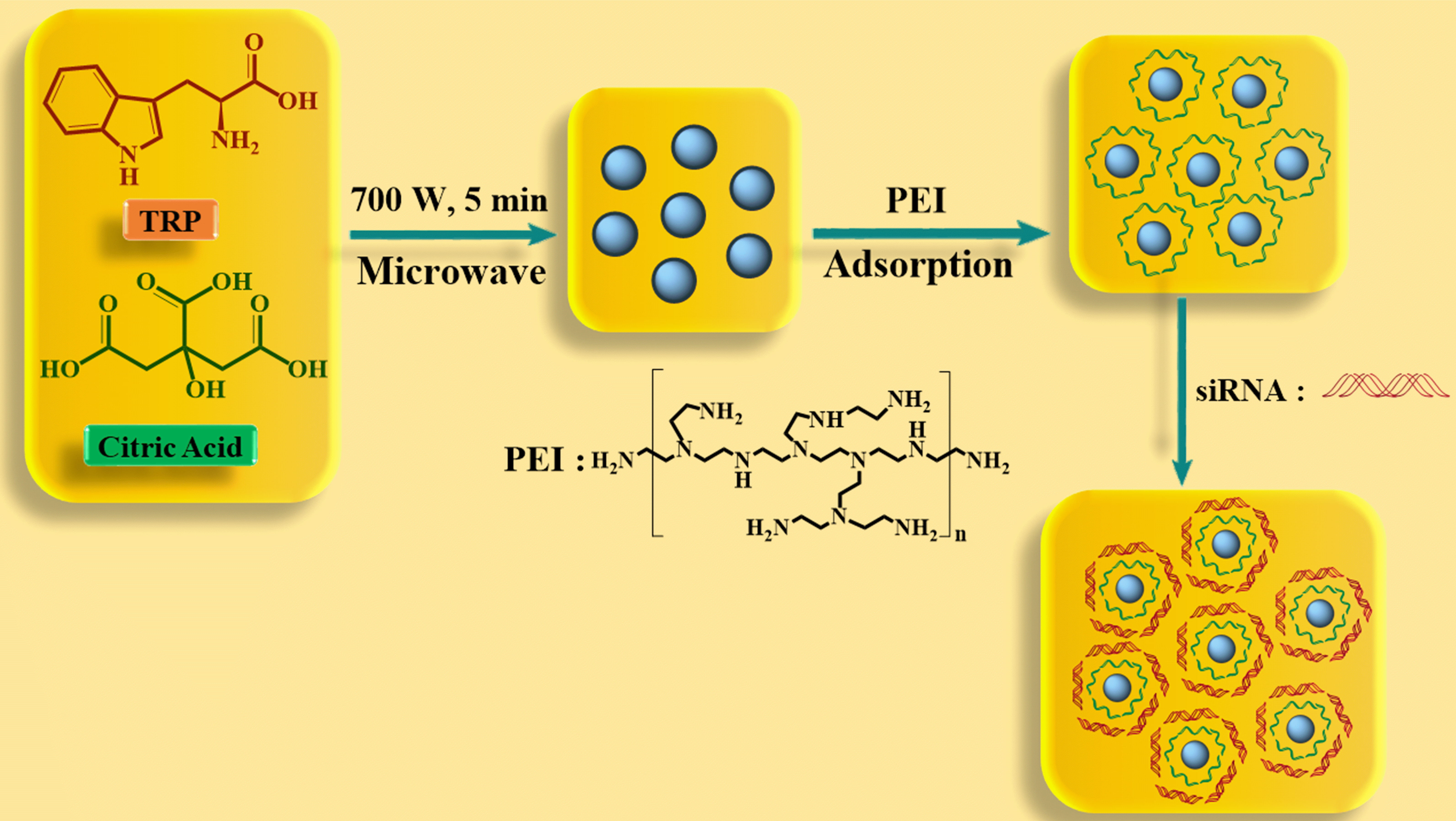
Diagram showing the creation of Cdots and the Cdots-based nanocarrier for siRNA delivery.
Quantum dots (QDs) offer a biocompatible and optically fluorescent surface that can serve as a versatile platform for gene therapy delivery. A biocompatible water-soluble QD was designed and functionalized on the surface with plasmid DNA, making it suitable for direct application in gene therapy. To regulate the timing of protein expression, this method employs chemoselective coupling chemistry between an InP/ZnS quantum dot (QD) and a linker DNA (DNAlinker). The QD-DNAlinker was employed to condense linear DNA (lDNA) containing the CMV promoter and DsRed-Express gene on its surface. Following transfection of the QD-lDNA into Chinese Hamster Ovary (CHO) cells, analysis of DsRed-Express expression through optical and flow cytometry methods showed delayed protein expression for both coupling chemistries compared to naked lDNA. The results indicate that QD-S-lDNA produces proteins more quickly than QD-NH-lDNA. Their analysis suggests that the protein expression delay can be attributed to the specific site of coupling between the QD and DNAlinker, as well as its effect on the strength of lDNA packing. The NH-DNAlinker is thought to couple to the facets through a condensation reaction, while the S-DNAlinker is hypothesized to couple via direct exchange at the vertices of the QDs. The protein expression delay is a consequence of the slower exchange rate at the facets compared to the vertices. The ability to regulate coupling chemistry and release timing from the QD surface offers a means for dose control in transient gene therapeutics. Moreover, it underscores the potential of QD delivery strategies as multifunctional, targeted drug-carrying platforms capable of concurrently regulating dosing. To visualize the effect of functionalization on the surface of the QD along with toxicological effects within the cells, researchers mainly focused on HIV cell-penetrating peptide functionalization as well as on “Trans-Activator of Transcription” (TAT). Utilizing optical microscopy, the cellular uptake of both 10% TAT and 100% TAT passivated QDs is observed to exhibit swift and widespread accumulation throughout the cell cytosol (Fig. 9). The impact of these substances on necrosis, apoptosis, and metabolic damage in CHO cells were examined in toxicity studies using flow cytometry. According to this research, the toxic effects of the cell-penetrating QDs depend on both the concentration of QDs added and how much CAAKA-TAT is applied to the QD’s surface. These findings support the suitability of QDs as nanoscale, self-transfecting delivery platforms for gene or drug therapy [43].
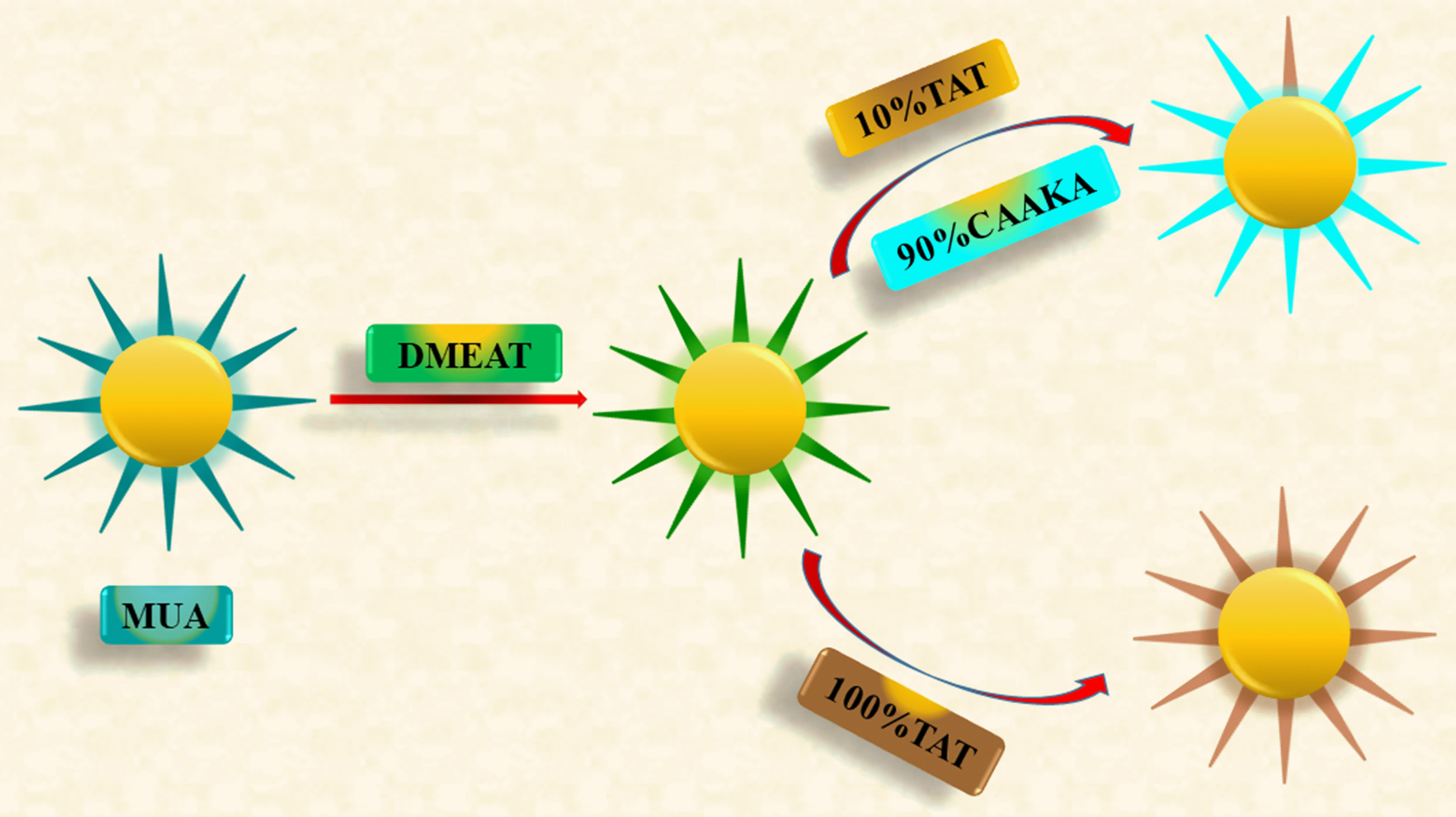
Diagram illustrating the synthesis of both QDs passivated by 100% CAAKA-TAT and QDs co-passivated by 10% CAAKATAT and 90% CAAKA.
Parkinson’s disease (PD) is a prevalent neurodegenerative condition characterized by the gradual loss of dopaminergic neurons in the substantia nigra pars compacta, leading to a decline in dopamine levels in the brain. Levodopa (L-DOPA) and dopamine agonists are two medications used to treat Parkinson’s disease, providing temporary release from symptoms during the early stages of the disease. Nevertheless, it is challenging to halt or slow the neurodegenerative progression of PD by utilizing these treatment approaches. As a result, there is a pressing need to develop innovative and more effective treatments for PD.
To cure Parkinson’s disease (PD), in 2022, Lin et al. [44] created a unique brain-targeted ZnO quantum dots (QDs) nanoplatforms that deliver genes into the brain to interfere with SNCA expression. A composite containing nerve growth factor (NGF) graft and glutathione (GSH)-modified ZnO QDs was developed and employed for gene delivery in the treatment of Parkinson’s disease (PD) models (Fig. 10). TEM images of spherical ZnO@Polymer and ZnO@Polymer-NpG particles showed an average size of around 5 nm. Additionally, by using DLS, the average hydrodynamic size of ZnO@Polymer and ZnO@Polymer-NpG was determined to be approximately 10 nm. The fluorescence tracking data revealed that the ZnO QDs composites were capable of delivering genes into the brain, crossing the blood-brain barrier (BBB), and subsequently releasing genes through lysosomal escape. Moreover, both In-vitro and In-vivo experiments demonstrated the neuroprotective effects of the ZnO QDs composites laden with interfering genes and nerve growth factor (NGF) in treating Parkinson’s disease. The treatment efficiently reversed the neurodegenerative process with minimal toxicity. Investigation of the toxic effects of ZnO QDs composites on organs apart from the brain was done using histopathological examination. After receiving treatment, no discernible pathological abnormalities were found in the mice’s major organs, according to the hematoxylin and eosin (H&E) staining data. Additionally, routine blood testing revealed that the particles had no appreciable inflammatory effect on PD mice. The findings indicate that ZnO@Polymer-NpG exhibited favorable biocompatibility when tested In-vivo. By integrating these versatile ZnO QDs nanoplatforms, they present a promising theranostic approach to Parkinson’s disease treatment [44].
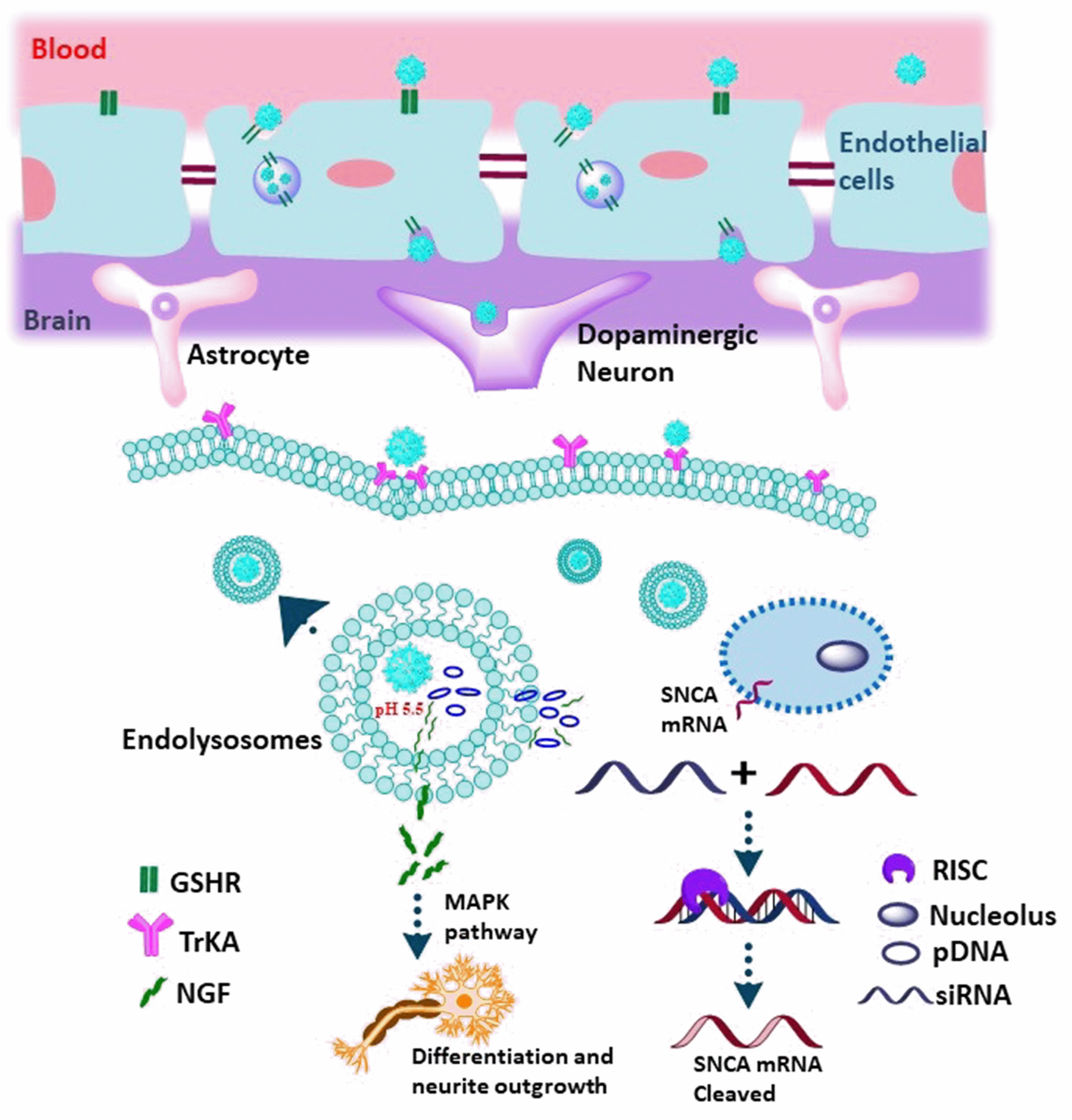
Schematic representation of the ZnO@Polymer-NpG therapy for
Parkinson’s disease. As a brain-targeted ligand that penetrates the BBB, GSH
binds to cell surface receptors on brain endothelial cells and then facilitates
the transcytosis of nanoparticles across the cell layer. When ZnO@Polymer-NpG is
internalized, lysosomal escape causes the release of NGF and pDNA, which creates
siRNA to silence the cellular SNCA (
In 2012 and 2014, Liu et al. [45] and Wang et al. [46], respectively, introduced Carbon Quantum Dots (CQDs) as nanocarriers capable of In-vitro and In-vivo gene delivery, with the unique feature of being trackable for imaging purposes.
Liu et al. [45] in 2012, by pyrolyzing glycerol and branched PEI25k in a single step with microwave assistance, carbon nanoparticle formation and surface passivation were carried out simultaneously, yielding polyethyleneimine (PEI) functionalized carbon dots (CD-PEI) (Fig. 11). The presence of the cationic polymer layer on the surface of the nanocarrier is expected to aid in the transfection of plasmid DNA, while the embedded carbon dots that exhibit visible fluorescence properties can serve as effective agents for bioimaging. Here, three CD-PEIs (CD-PEI-A, CD-PEI-B, and CD-PEI-C) were designed by varying microwave irradiation times. Elementary analysis of the nanoparticles reveals that they are composed of CD-PEI-A containing 47.53% carbon, 24.71% nitrogen, 9.82% hydrogen, and 17.94% oxygen. CD-PEI-B includes 54.13% carbon, 22.93% nitrogen, 9.41% hydrogen, and 13.53% oxygen. High-resolution transmission electron microscopy (HRTEM) images show that the particle size ranges from 4 to 12 nm. To determine the surface functional groups of the CD-PEIs, Fourier Transform Infrared Spectroscopy (FT-IR) spectra were examined. It was established that this CD-PEI is water soluble and relies on excitation wavelength to emit stable, bright multicolor fluorescence. All CD-PEI samples were found to be capable of condensing DNA at a very low weight ratio by agarose gel electrophoresis assay. All three CD-PEIs can condense DNA into nanoparticles (below 50 nm), which is advantageous for effective cellular transport, according to HRTEM pictures of the CD-PEI/pDNA complexes. It was found that in comparison to control PEI25k, CD-PEIs obtained at the proper pyrolysis time displayed less hazardous and greater or equivalent levels of plasmid DNA gene expression in COS-7 and HepG2 cells. Achieving the optimal performance of the CD-PEI vector required careful control of the pyrolysis time to strike a balance between C-dot generation and PEI degradation. The multicolor fluorescence of the C-dot/DNA complexes was vividly visible in the cytoplasm, illuminating CD-enormous PEI’s potential for bioimaging and biolabeling [45].
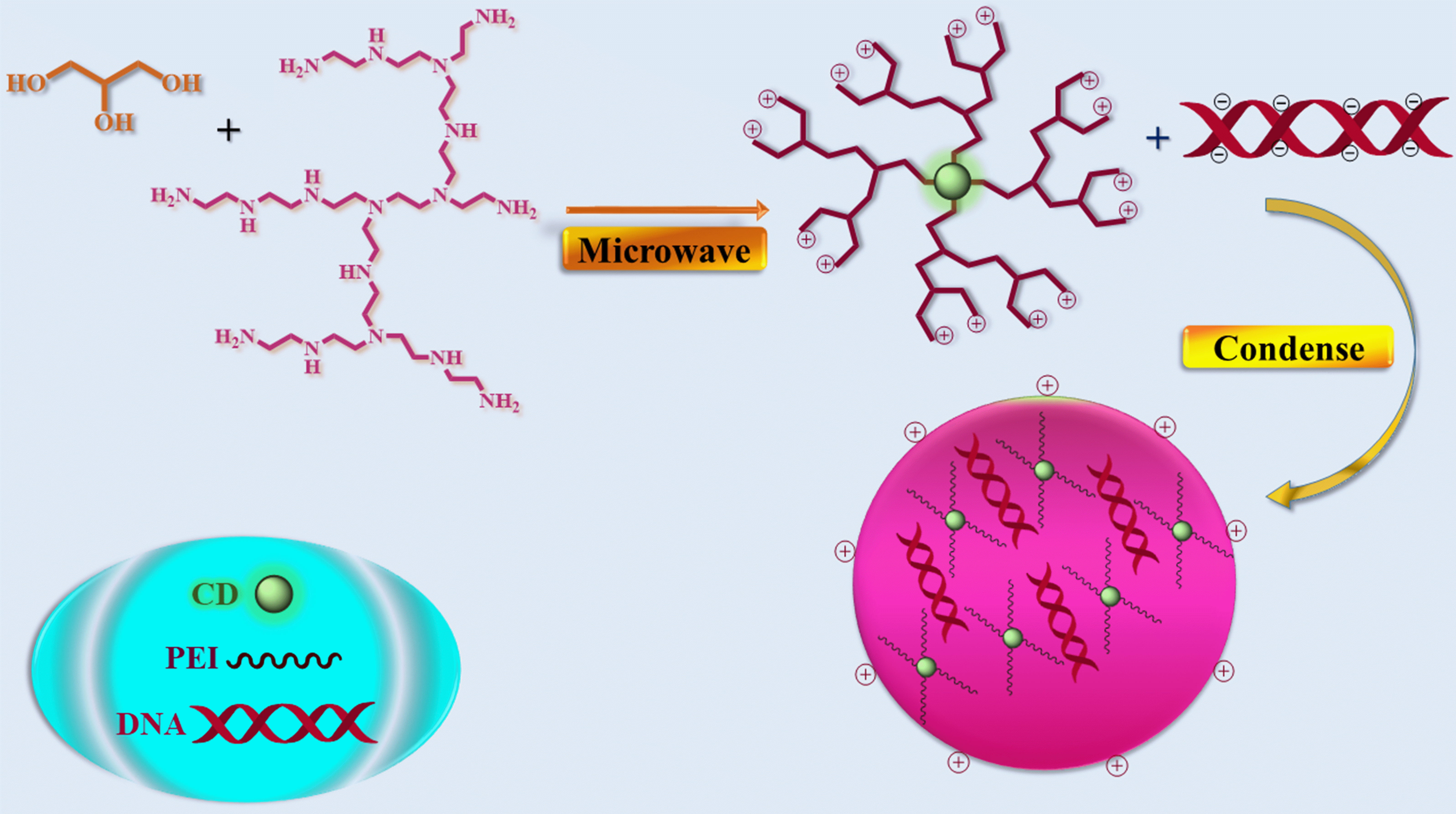
Illustration of the CD-PEI and CD-PEI/pDNA complex formation. CD, Carbon Dot.
In 2014, using Alkyl-PEI2k for surface passivation, Wang et al. [46] successfully created and characterized a novel class of photonic Cdot-based gene delivery nanocarrier. Alkyl-PEI2k was employed for both surface passivation and gene delivery to create Alkyl-PEI2k-Cdots (Fig. 12). According to their research, the nano vector was found to exhibit uniformity with a narrow size distribution, favorable stability, and photoluminescent properties. The Alkyl-PEI2k-Cdots sample was examined using scanning transmission electron microscopy (STEM), which revealed that the Alkyl-PEI2k-Cdots sample displayed excellent dispersion, with individual Cdots having an average particle size of approximately 10 nm. The average height and length of the Cdots were determined using an atomic force microscope (AFM) and found to be roughly around 15 nm. In addition, through agarose gel electrophoresis, fluorescence performance analyses, and toxicity assessments, it was observed that Alkyl-PEI2k-Cdots exhibit stable binding, protection, and efficient delivery of siRNA and plasmid DNA (pDNA), making them promising candidates for gene therapy applications due to their retained fluorescence properties and high biocompatibility. In general, PEI’s molecular weight substantially correlates with its transfection effectiveness and cytotoxicity. In contrast to lesser molecular weight PEI, like PEI2k, which has less cytotoxicity, PEI-2k is an extremely effective gene transfection agent. An MTT cell viability assay was used in the current work to measure the cytotoxicity of Alkyl-PEI2k-Cdots following transfection treatment. According to the results, even at the greatest N/P ratio, the Alkyl-PEI2k-Cdots/siRNA complexes cytotoxicity remained relatively low compared to other control groups. The low molecular weight and low density of amino groups in Alkyl-PEI2k may be responsible for the complexes’ low cytotoxicity. They believe that Alkyl-PEI2k-Cdots, which have minimal cytotoxicity, efficient gene delivery, and fluorescent features, offer a safe and effective route for gene therapy and optical molecular imaging based on this methodical research [46].
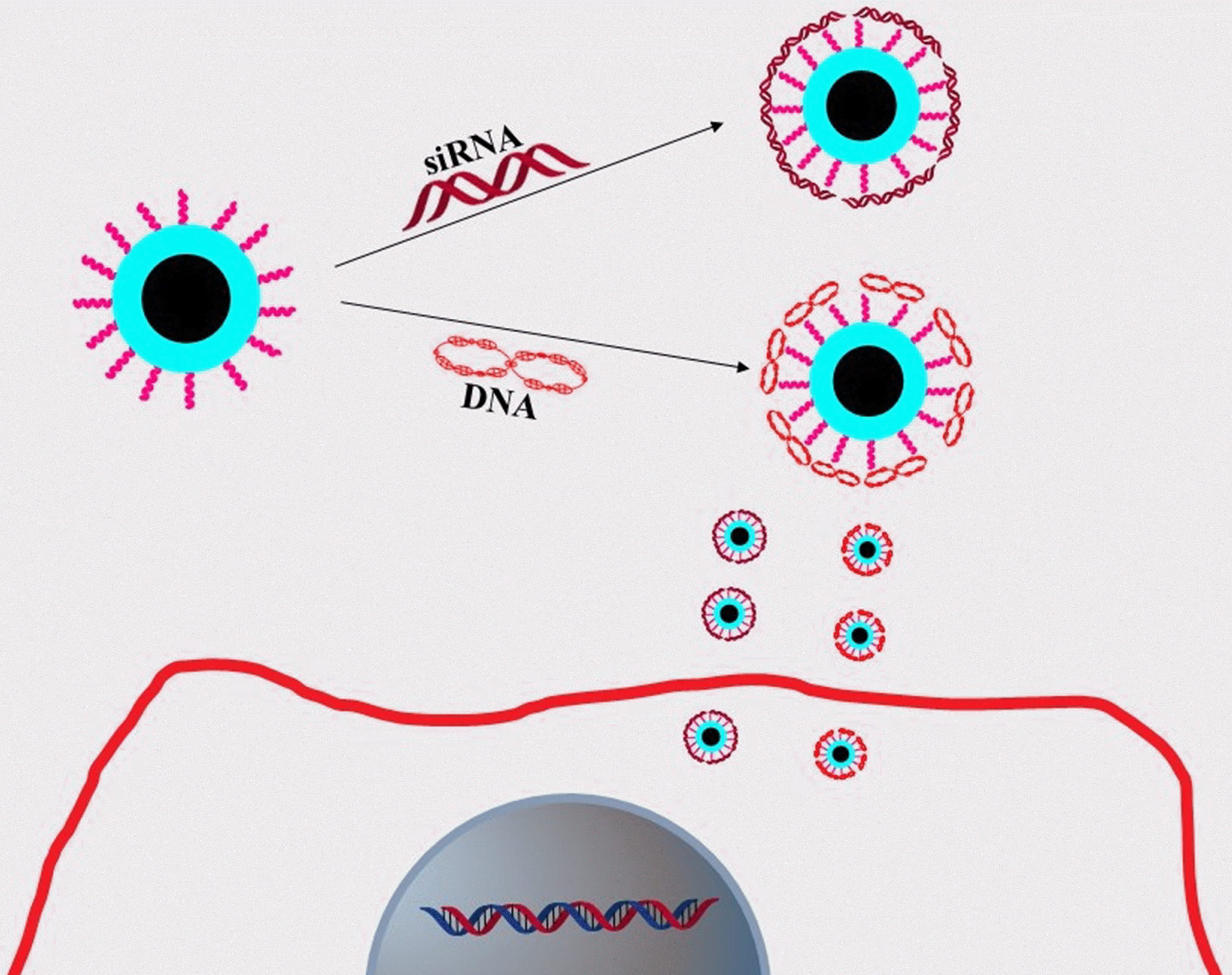
Diagram showing the creation of Alkyl-PEI2k-Cdots/siRNA complex and delivery into cancer cells.
Once more, a successful gene therapy process depends on identifying the therapeutic gene, delivering its effective delivery into the nuclei of specific cells, and accurately monitoring its presence and activity. For gene therapy, a good nanocarrier must be highly effective, free of cytotoxicity and immunogenicity, able to carry the gene and safeguard it from deterioration, cross biological barriers, and deliver the gene. Non-viral vectors, such as cationic lipids and polypeptides, have gained attention as safe alternatives to viral vectors, as they do not exhibit negative side effects like cytotoxicity and immunogenicity. As a result, significant research is being done to discover new non-viral nanocarriers that will effectively deliver therapeutic genetic tools into desired cells. Fusion nano-peptides, acting as versatile carriers, play a crucial role in gene transfer into cells by efficiently condensing DNA, rupturing endosome membranes, and promoting DNA translocation to the nucleus.
In 2017, Ghafary et al. [47] developed non-toxic graphene quantum dots
(GQDs) (graphene quantum dots (GQDs) with non-toxic properties) using Hummer’s
method that emits two distinct colors (green and red). Through non-covalent
interactions, the MPG-2H1 chimeric peptide and plasmid DNA (pDNA) are conjugated
to the GQDs (Fig. 13). TEM was used to analyze the shape and size distribution of
both GQDs and their complexes. According to the results, the MPG-2H1/pDNA
complexes have an average size of 200 nm. The typical diameter of GQDs is
approximately 80 nm. The MPG-2H1/pDNA/GQDs complexes, as measured by DLS, have a
size range of 135–280 nm, with an increased
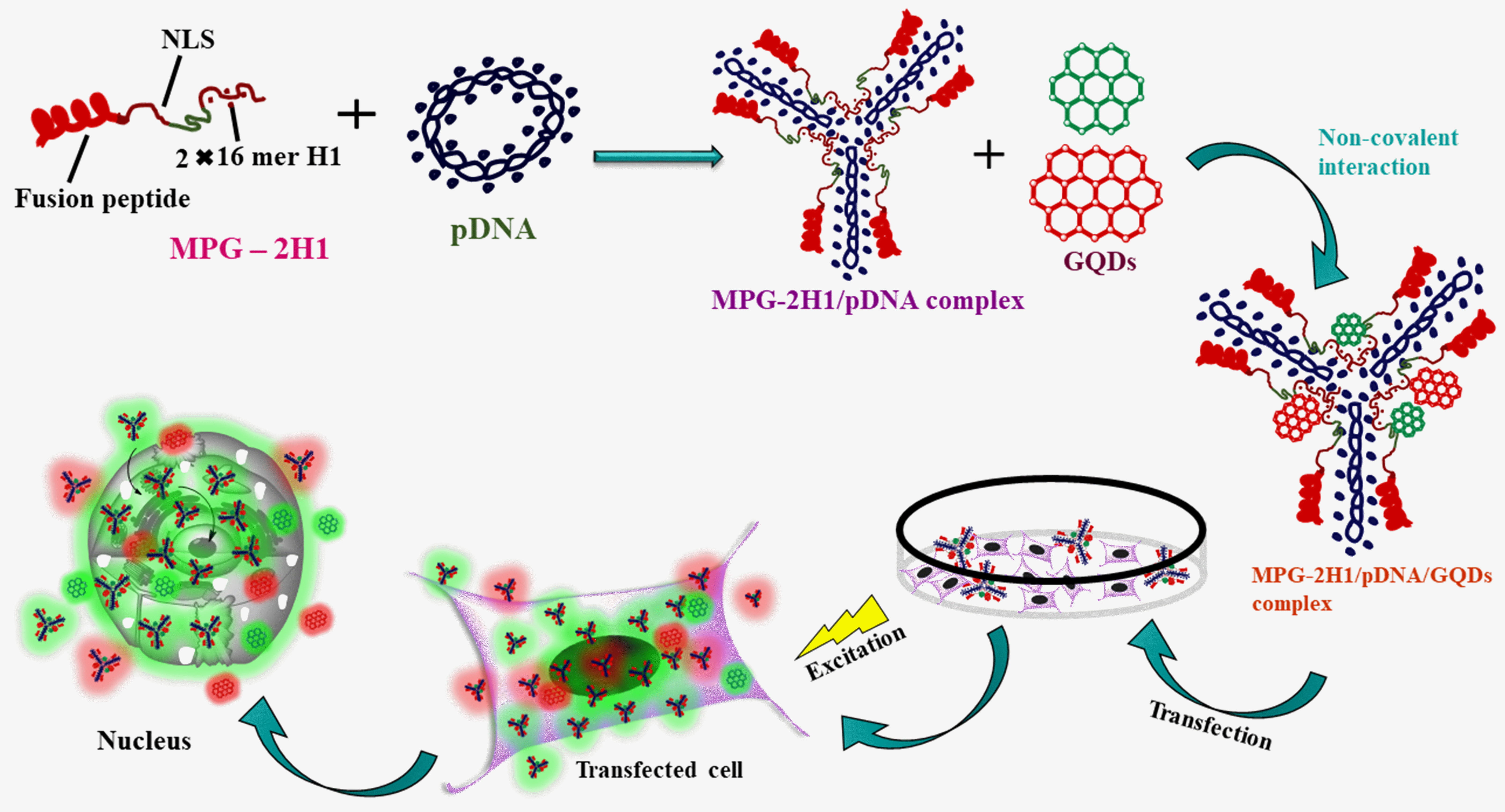
MPG-2H1/pDNA/GQDs complexes are assembled schematically by non-covalent interactions, transfection into cells, and excitation.
Features of Quantum Dots used for gene delivery and gene silencing are summarized in Table 2 (Ref. [25, 26, 27, 28, 39, 40, 41, 42, 43, 44, 45, 46, 47].
Sl. No | Quantum dots | Activity | |
1 | Proton-sponge-coated quantum dots were prepared by using amphipol PMAL, grafted with polyethyleneimine (PEI) as an encapsulation polymer. QD-PMAL-PEI nanoparticles [25]. | Prevention of glioma cell migration | |
2 | Used PEGlyated quantum dot (QD) core as a scaffold. siRNA and tumor-homing peptides (F3) were conjugated to functional groups on the particle’s surface [26]. | To treat metastatic cancer | |
3 | Proton-Sponge Coated Quantum Dots [27] | To assess and enhance the effectiveness of imaging and therapeutic nanoparticles | |
4 | L-Arg (L-arginine) and |
The use of siRNA delivery to silence the HPV18 E6 gene and facilitate intracellular imaging. | |
5 | CdSe/ZnS QDs with the conjugation of amino-polyethylene glycol (PEG) [39] | Treating neurodegenerative disorders like Alzheimer’s disease | |
6 | CdSe core, ZnS shell QDs [40] | For the targeted silencing of the survivin gene using RNA interference (RNAi) in Tca8113 human tongue cancer cells | |
7 | Cadmium sulphoselenide/zinc sulfide quantum dots (CdSSe/ZnS QDs) [41]. | Genetic therapy of glioblastoma. | |
8 | Cdots-based and PEI-adsorbed nanocarrier (Cdots@PEI) [42] | Delivery of siRNA in human gastric cancer cell line MGC-803 against antiapoptotic protein Survivin. | |
9 | InP/ZnS quantum dot (QD) [43] | Self-transfecting delivery scaffolds for gene or drug therapy. | |
10 | ZnO quantum dots [44] | Treating Parkinson’s disease | |
11 | Polyethyleneimine (PEI) functionalized carbon dots (CD-PEI) [45] | Gene delivery and bioimaging | |
12 | Alkyl-PEI2k-Cdots [46] | Surface passivation and gene delivery | |
13 | Graphene quantum dots (GQDs) using Hummer’s method [47] | Simultaneous Gene Delivery and Tracking |
The majority of experts concur that gene therapy represents a highly intriguing and promising application of DNA research to date. The ease of the process will determine how widely this therapy could be used. Scientists anticipate that within the next 20 years, gene therapy could potentially serve as the ultimate cure for all hereditary diseases. It is a developing field that necessitates multifunctional delivery platforms to overcome cellular barriers. It still faces difficulties due to the absence of a reliable delivery system that can administer stable small interfering RNA (siRNA) or DNA selectively to the target tissue region. siRNA or DNA is transported by a multifariousness of mechanisms, such as liposomes, polymers, peptides, virus-based vectors, etc. Since they are non-fluorescent, these carriers cannot be used to observe the siRNA or DNA distribution procedure. Quantum dots, due to their dual-modality optical and fluorescent characteristics, enabled real-time monitoring and precise ultrastructural localization of the QD-siRNA or QD/DNA complexes during delivery and transfection processes. Despite the difficulties in clinical translation, QDs are scientific advances that might revolutionize cancer diagnostics. Although it is clear that QDs-based In-vivo molecular imaging has benefits, their biosafety still has to be thoroughly investigated and assessed. Researchers are still concerned about the clearance of the consumed QDs from the system. Renal filtration and urine excretion are effective ways to remove quantum dots. Additionally, if the nanoparticles are not eliminated, and there is a means to remove them from the body, the toxicity of the nanoparticles should be reduced. QDs are currently used In-vitro for the detection of cancer-associated proteins, the capture of CTCs, and molecular pathology. These applications highlight the dynamic process of cancer formation and enable the creation of a new platform for improved comprehension of tumor heterogeneity. Nanotechnology has a clinical advantage for the detection, therapy, and prevention of cancer. Although the progress in developing quantum dot nano-transporters for pharmaceutical purposes has encountered unforeseen obstacles, this technology remains nascent. Nonetheless, quantum dots have shown significant utility and promise across diverse domains, underscoring their value in advancing scientific and medical applications for the benefit of humanity. The research studies discussed in this review article will provide a framework for designing efficient QD-based nanocarriers that can successfully carry therapeutic genetic tools into targeted cells. Following these discoveries, the researchers created some special QDs that successfully attached to the siRNA or DNA and delivered it to its intended site. The field of gene delivery may be advanced with the deployment of these QD-based delivery systems.
SM searched all the articles and prepared the figures, schemes, and manuscripts. JR guided throughout the review work. PS conceptualized the review and edited and thoroughly crosschecked the whole manuscript to make it presentable. All authors contributed to editorial changes in the manuscript. All authors read and approved the final manuscript. All authors have participated sufficiently in the work and agreed to be accountable for all aspects of the work.
Not applicable.
SM is sincerely thankful to Visva-Bharati, India for giving her the research facilities. SM also acknowledges Shrabani Saha and Diptiman De for helping her throughout the review work.
This research received no external funding.
The authors declare no conflict of interest.
Publisher’s Note: IMR Press stays neutral with regard to jurisdictional claims in published maps and institutional affiliations.