- Academic Editor
Background: Interspecies variations in mammalian red blood cells (RBCs)
are observed in circulating RBC lifespan, cell size, fluidity, aggregation, water
permeability, metabolism, lipid composition, and the overall proteome. Bovine RBC
cell membrane is deficient in phosphatidylcholine and exhibits anomalies in the
arrangement of phosphatidylethanolamine within the lipid bilayer. However, like
human RBCs, virtually all the aminophospholipid phosphatidylserine (PS) is found
within the cytoplasmic side of the cell membrane of intact circulating bovine
RBCs. During apoptotic cell death of human and murine RBCs, PS translocates to
the outer leaflet of the cell membrane via Ca
Evolutionary differences in the circulatory systems of vertebrates are paralleled by functional variations in red blood cell (RBC) morphology, numbers, and biophysical properties [1, 2]. Mammalian RBCs are characterized by enucleation of erythroid cells towards the end of their maturation [3]. Interspecies differences in mammalian RBCs are observed in RBC cell size, aggregation, metabolism, membrane lipid composition, and the overall proteome [4, 5, 6, 7, 8]. These variations dictate differences in hemorheological properties and the RBC’s ability to adapt to various extracellular environmental challenges in the circulation [9, 10, 11, 12]. RBC lifespan varies across mammalian species with bovine RBCs known to circulate longer (~160 days) than human RBCs (~120 days) [13]. Premature cell death of circulating RBCs prior to completion of their expected lifespan in vivo has been demonstrated in a wide range of human diseases associated with anemia, as well as in mouse models of different clinical conditions [14]. However, little is known about this phenomenon in cattle.
During their lifespan, RBCs accrue a wide range of physical injuries together
with enzymatic alterations leading to their senescence and subsequent clearance
from the circulation by phagocytic catabolism [13, 14, 15]. In the context of various
systemic diseases in humans, RBCs may be afflicted by oxidative injuries leading
to reduced survival [16]. Other pathophysiologic cell stressors, such as
fever-range hyperthermia, extracellular tonicity alterations, and energy
starvation, can trigger RBC dysfunction and premature cell death [14]. When
excessive loss of RBCs is not compensated by enhanced erythropoiesis in the bone
marrow, anemia ensues [14]. Apoptotic cell death in RBCs, also known as
eryptosis, is characterized by the breakdown of the phospholipid asymmetry in the
cell membrane leading to the flipping of the aminophospholipid,
phosphatidylserine (PS), towards the exterior leaflet of the cell membrane [14, 17]. While a wide array of mechanisms are operative during RBC cell death,
Ca
Structurally, ruminant RBCs exhibit anomalies in their cytoplasmic membrane phospholipid architecture as compared to RBCs from other mammalian species such as the presence of N-acylated aminophospholipids [18]. Bovine RBCs are deficient in phosphatidylcholine and possess high levels of sphingomyelin in the cell membrane [19]. Strikingly, unlike human RBCs, the outer leaflet of bovine RBC cell membrane is reported to have a significantly lower proportion of phosphatidylethanolamine (2% versus 20%) [19]. Differences in composition of the lipid membranes of bovine and human RBCs have been linked with differential RBC membrane fluidity [20] and water permeability [21] between the two species. Despite the unique lipid organization in the bovine RBC membrane PS is found entirely in the inner leaflet of the lipid bilayer of intact cells, like human RBCs [19].
In bovine RBCs, the possibility of PS translocation to the outer leaflet in response to proapoptotic signals, as in human and murine RBCs, remains elusive. One important clue on the clinical significance of apoptotic cell death in stressed bovine RBCs comes from a previous study showing increased RBC PS expression in anemic cattle infected with the intraerythrocytic parasite, Theileria sergenti [22]. Infected cattle have been reported to display enhanced clearance, hemolysis, and morphological changes in RBCs [22, 23, 24]. Systemic bovine diseases involving RBC dysfunction and anemia can influence the overall health and productivity of livestock. Thus, characterization of the apoptotic cell death process in bovine RBCs would provide novel insights into the pathogenesis of bovine anemia resulting from accelerated loss of circulating RBCs.
Bovine blood was procured from a local abattoir (Windcrest Meat Packers Ltd.,
Port Perry, ON, Canada). Cow blood was collected into an anticoagulant-containing
tube immediately following an incision in the neck as described previously [25].
For bovine RBC isolation, whole blood was centrifuged for 10 min at 1000
Cellular markers of RBC apoptosis were examined using flow cytometry (BD Accuri
C6 Plus) analysis in the FL1 channel (excitation wavelength of 488 nm and an
emission wavelength of 530 nm) following previously described protocols [26]. RBC
PS expression was quantified using annexin V FITC (1:100 dilution; BioLegend, San
Diego, CA, USA; verified reactivity with PS in all mammalian species according to
manufacturer). Relative intracellular Ca
For the determination of osmotic resistance, 2 µL of RBCs were added to 1000 µL of different mixtures of isotonic PBS and hypotonic distilled water with varying osmolalities. Relative hemolysis was measured in terms of absorption measured at 405 nm as described previously [26].
Data are expressed as means
Firstly, we examined the effects of artificially enhancing intracellular
Ca
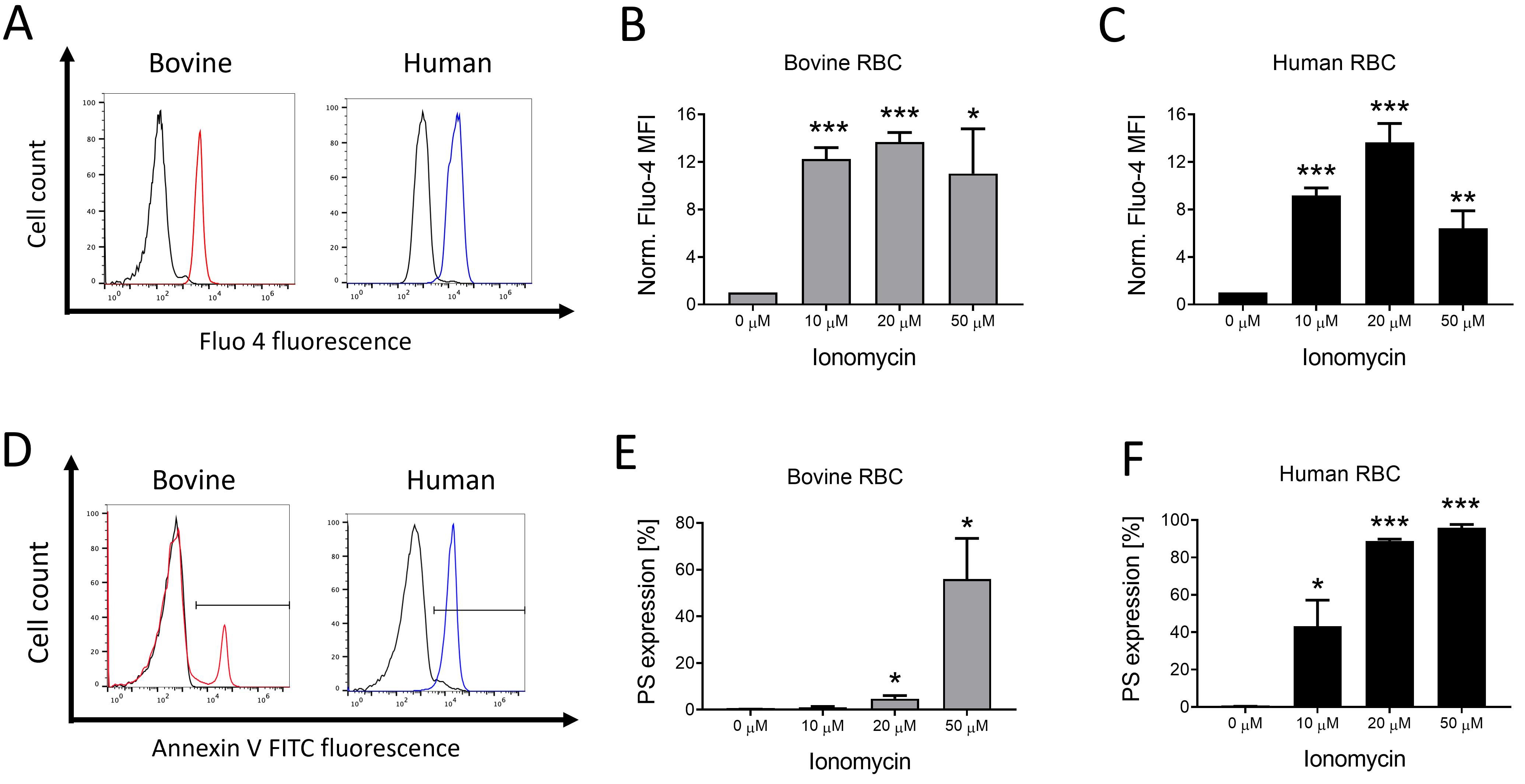
Ionophore-mediated Ca
Oxidative damage to human RBCs is paralleled by increased cation channel
activation leading to increased Ca
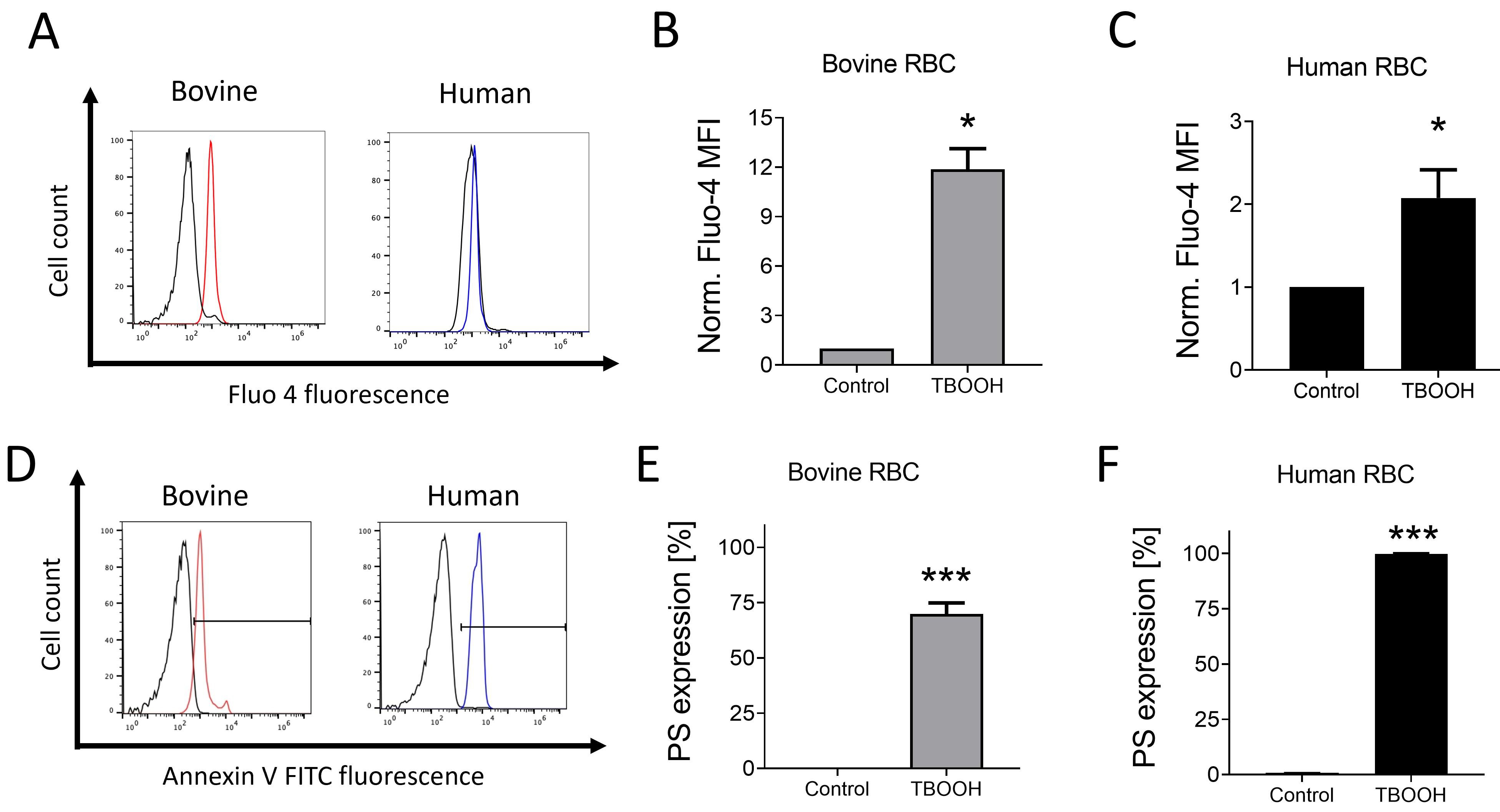
Oxidative stress stimulates Ca
Next, we evaluated bovine and human RBC survival under the conditions of
fever-grade hyperthermia and glucose deprivation. As shown in Fig. 3A–C, a
72-hour incubation of bovine RBCs in Ringer’s solution, glucose-devoid
conditions, or exposure to 41 °C temperature upregulated intracellular
Ca
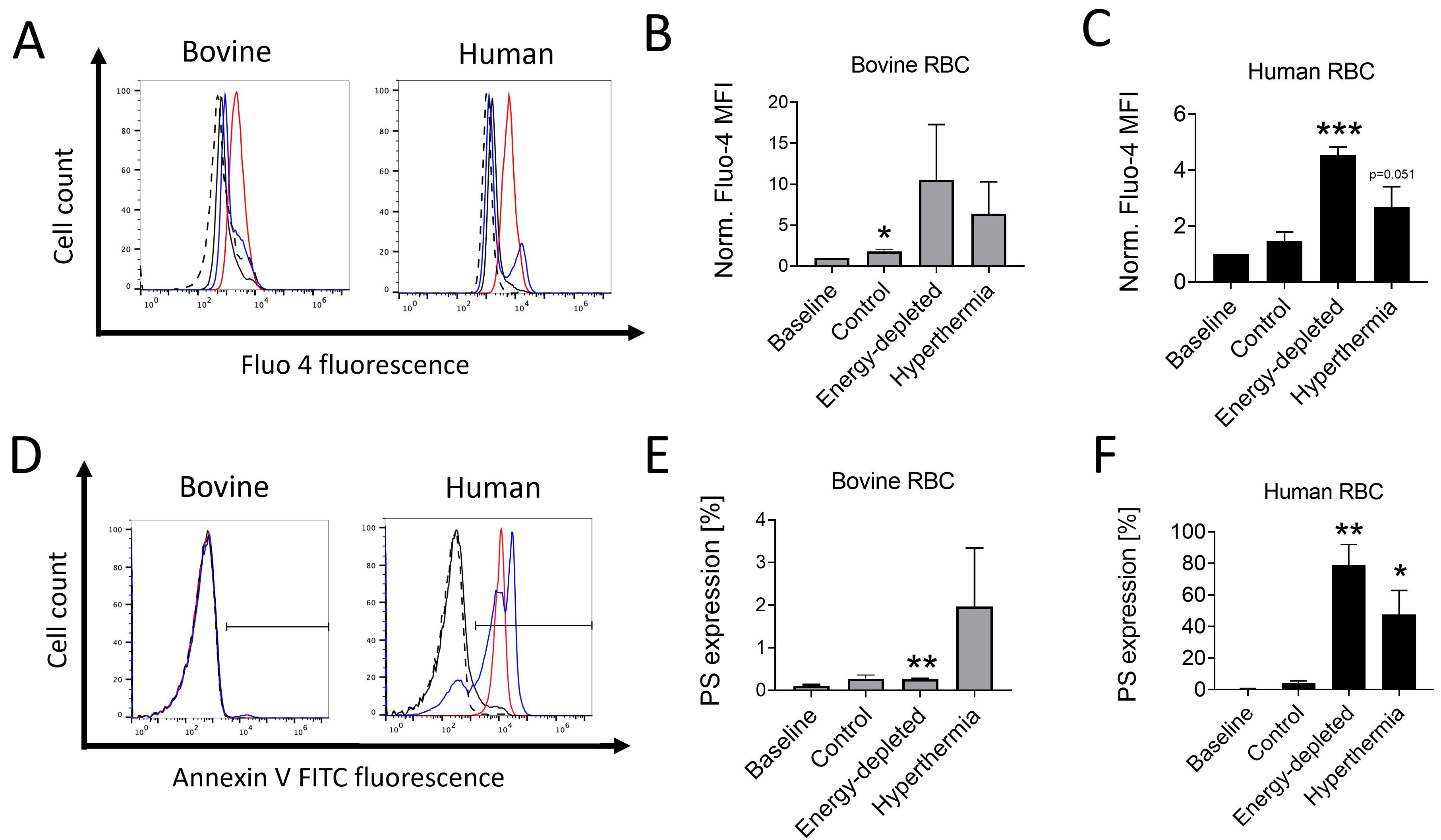
Effects of hyperthermia and energy starvation on cytoplasmic
Ca
Additional experiments were conducted to assess whether fever-grade hyperthermia or energy starvation induced oxidative stress in bovine and human RBCs. As shown in Fig. 4A–C, DCF fluorescence, indicating increased ROS levels, was significantly enhanced in both bovine and human RBCs after a 72-hour incubation, under glucose-devoid conditions or incubation in hyperthermic conditions (41 °C), suggesting that despite starvation- and hyperthermia-associated redox imbalances in RBCs from both species, bovine RBCs displayed a minimal increase in membrane PS expression.
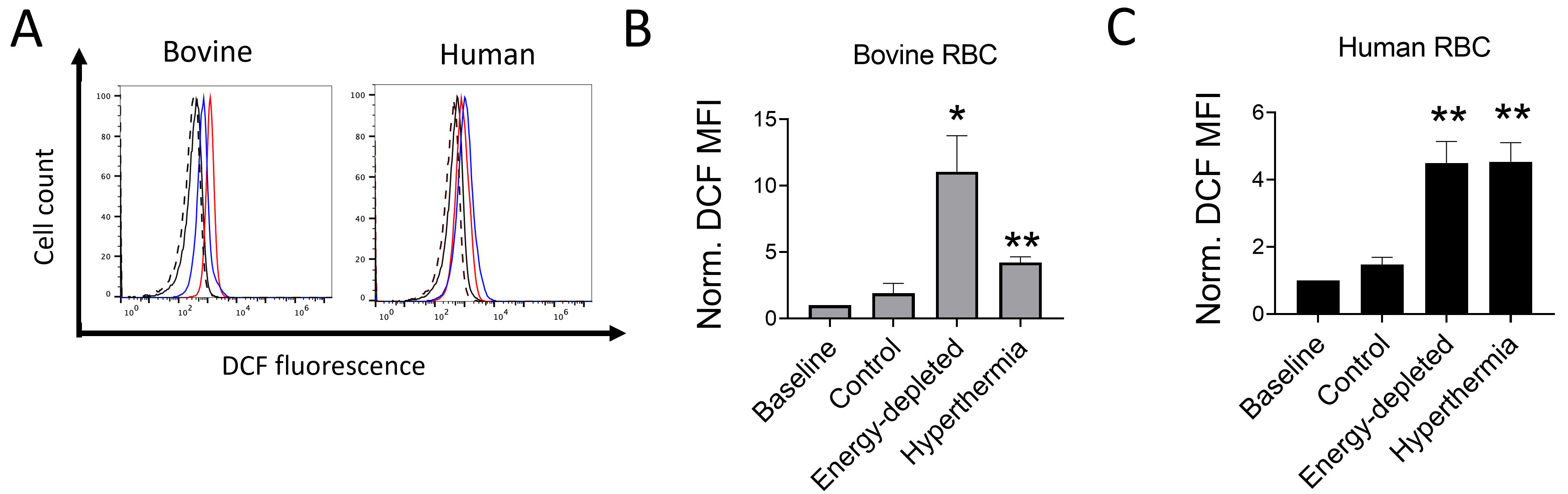
Effects of hyperthermia and energy starvation on reactive oxygen species (ROS) production
in bovine and human RBCs. Representative histogram (A; Dotted: Baseline; Black:
Control; Red: Energy-depleted; Blue: Hyperthermia). Means
Deviations in extracellular tonicity are known to trigger ionic and fluid shifts in RBCs. Hemolysis in bovine RBCs was significantly higher at 30–50% reductions in osmolality as compared to human RBCs (Fig. 5A). Hypertonicity (addition of 600 mM of sucrose into the medium) significantly enhanced PS expression in human RBCs after 4 hours (Fig. 5B,E). In contrast, a modest but significant increase in PS exposure was observed in bovine RBCs after a 48-hour, but not 4-hour, incubation in hypertonic Ringer solution (Fig. 5B–D) suggesting that bovine RBCs are relatively more resistant to hypertonic stress-induced cell death as compared to human RBCs.
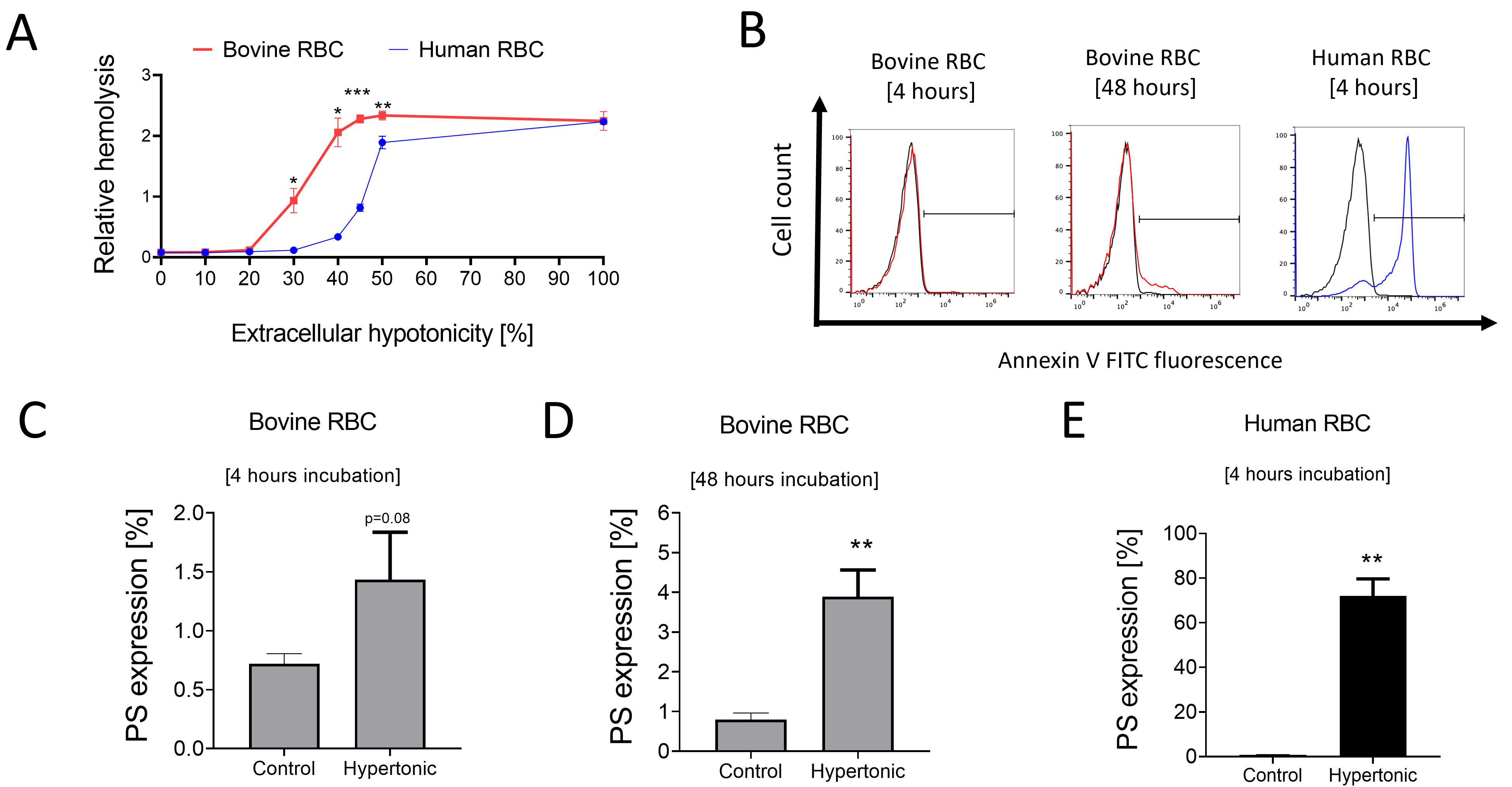
Impact of extracellular osmolality changes on bovine and human
RBCs. Means
Clinically, the pathogenesis of anemia in cattle has been ascribed to various factors such as immune-mediated RBC destruction [29], parasitic infections [30, 31, 32], and nutrient deficiency [33, 34]. In vitro and in vivo studies provide some mechanistic evidence that increased erythrophagocytosis and extravascular RBC catabolism are responsible for Trypanosoma-elicited anemia, a common parasitic infection in cattle [35, 36]. Despite the morphological and compositional peculiarities of bovine RBCs, our in vitro data indicate that bovine RBCs undergo a cell death process with some mechanistic similarities to that observed in human [14], murine [14], and canine RBCs [37].
Supraphysiologic Ca
Heat stress in cows can affect their physiological homeostasis and reduce livestock productivity [41]. Cattle suffering from some types of infectious febrile conditions such as anaplasmosis and trypanosomiasis, often display anemia [42, 43]. Normal bovine body temperatures have been reported to vary between 38–39.5 °C depending on age and breed [44]. Our data show that exposure of bovine and human RBCs to hyperthermia increased ROS levels. However, bovine RBCs showed a minimal increase in PS expression despite prolonged hyperthermia exposure, suggesting that bovine RBCs potentially have a higher acute adaptability, possibly underpinned by interspecies metabolic variations, to hyperthermic challenge. Interestingly, exposure of bovine RBCs to extracellular hypertonic stress for relatively shorter periods did not elicit increased PS exposure as compared to human RBCs and only a prolonged incubation of bovine RBCs modestly enhanced PS expression. However, in contrast to human RBCs, bovine RBCs displayed markedly increased hemolysis in response to reduction in extracellular osmolality. Indeed, RBCs from cows have previously been reported to display higher levels of hemolysis in response to various challenges compared to RBCs from various mammalian species [5, 45]. A limitation of the present study is that the relatively small sample size did not permit us to determine the effects of biological variables such as age, sex, and cow breed. Notably, age, sex, and genetic heterogeneity have been documented to influence RBC phenotype in other mammalian species [46, 47, 48]. Nevertheless, the strength of our study is that it provides the first published evidence of the cell death process in bovine RBCs and paves the way for follow-up studies in the future, relevant to veterinary medicine and the livestock industry.
From our data and previous findings taken together, it may be conjectured that structural and metabolic variations between human and bovine RBCs confer differential responses to stress-induced cell death patterns. Accordingly, further studies are required to fully discern the cell death machinery in bovine RBCs, which may potentially be an important mechanism underlying bovine anemia.
Data supporting the findings of this study are available from the corresponding author upon reasonable request.
BK and SMQ designed the experiments. BK, SM, and TM performed the acquisition and analysis of data. BK, SM, and SMQ wrote the manuscript and prepared the figures. RB, TES, HJT, JGJ, and SMQ advised on the research, provided resources, and/or interpreted the data. All authors have sufficiently participated in the research, reviewed the manuscript draft, edited the changes, approved the final version, and agreed to take responsibility for this work.
Use of bovine and human blood for research was cleared by Ontario Tech University’s Biosafety committee (permit# BSC28). RBC concentrates were supplied by CBS Blood4Research (CBS REB #2022.034).
The authors thank Masoomeh Eghtedari and Shivani Patel for their technical support, and Windcrest Meat Packers Ltd. staff (Port Perry, ON) for providing bovine blood samples.
SMQ received research funding from the Natural Sciences and Engineering Research Council of Canada (NSERC; RGPIN-2022-04382). RB was supported by a grant from the German Research Foundation (BI 2149/2-1). SM and TM were recipients of the NSERC Undergraduate Student Research Award.
The authors declare no conflict of interest.
Publisher’s Note: IMR Press stays neutral with regard to jurisdictional claims in published maps and institutional affiliations.