- Academic Editor
Mesenchymal stem cells (MSCs) offer great potential for use in stem cell-based therapies due to their unique regenerative potential via reconstructive and paracrine capacities. These therapies offer new hope for patients suffering from conditions that have no cure. Currently, mesenchymal stem cells (from adipose tissues, bone marrow, and umbilical cords) are most interesting for application in those therapies. Nevertheless, the development of MSC-based medical products requires thorough research and standardization that maximizes the therapeutic effect while minimizing side effects. One of the interesting novel approaches to achieving this goal is combining MSC therapy with an electromagnetic field (EMF). Many studies have shown that EMF can enhance the regenerative properties of MSCs by influencing stem cell fate through modulating differentiation, proliferation, cell cycle regulation, metabolism, and cytokine and growth factor secretions. Combination therapy of EMF-MSCs is a promising perspective; however, it is important to select appropriate EMF parameters to obtain beneficial therapeutic effects. Therefore, understanding the mechanisms involved in the EMF impact on MSCs is crucial. In this study, we provide an overview of the effects of EMF on the biological response and “fate” of MSCs, paying attention to the gaps in research that remain unfilled and discuss the clinical application of this approach.
The development of science over the previous decades has added to the understanding of the biological basis involved in many diseases and their pathogenesis, which, together with technological breakthroughs, enables the development of more effective and precise treatment methods. Regenerative medicine is a relatively new branch of medicine, which is an interdisciplinary field that deals with the development of treatment techniques aimed at restoring health through the optimization and implementation of methods that ensure the restoration and/or replacement of damaged cells, tissues, and organs [1, 2].
Stem cell-based therapy, as a branch of regenerative medicine, provides a potential therapeutic option via stem cells and their unique properties, such as an ability to self-renew and the potential to differentiate. Stem cell-based therapies bring new hope to patients with incurable diseases and disorders. Stem cells can be categorized by their differentiation potential (totipotent, pluripotent, multipotent, and unipotent) or by their source of origin (embryos, adult tissues, or reprogramed somatic cells) [3, 4]. Currently, the greatest interest for use in regenerative therapy is induced pluripotent stem cells (iPSCs) and adult stem cells [1, 4]. The iPSCs have great potential because of their pluripotency and lack of ethical concerns (in contrast to pluripotent embryonic stem cells). However, they have higher tumorigenic potential than multipotent stem cells, which is one reason (next to their unique advantages) why multipotent mesenchymal stem cells (MSCs) are currently most widely used in clinical and preclinical studies [5].
MSCs—first discovered by Friedenstein et al. [6] in bone marrow—are
a type of stem cell found in adults that are of great interest in regenerative
medicine owing to their unique properties, which offer potential use in stem
cell-based therapies [1, 7]. Importantly, the therapeutic application of MSCs is
based on their reconstructive effect on damaged cells and tissues and relates to
their differential properties. However, their paracrine function and ability to
secrete cytokines, enzymes, growth factors, and chemokines can also promote
regeneration processes [4, 7]. To standardize the concept of applying MSCs in
regenerative medicine, the International Society for Cellular Therapy (ISCT)
published a minimum criteria for defining MSCs. According to the ISCT, they
should be plastic-adherent and capable of differentiation into chondroblasts,
osteoblasts, and adipocytes in vitro as well as express specific surface
markers
The regenerative potential of MSCs has been proven by many research groups. When using MSCs in stem cell-based therapy, it is extremely important to standardize and optimize procedures that maximize the therapeutic effect, while concurrently minimizing side effects. One intensively researched tool to enhance stem cell-based therapy is electromagnetic fields (EMFs), which have been shown to impact MSC biology by influencing proliferation, symmetric and asymmetric cell division, differentiation, cell cycle progression, metabolism, and cytokine and growth factor secretions [9, 10, 11, 12, 13]. EMFs are areas of energy in the form of a combined effect of magnetic and electric fields. It can be grouped into two categories, which are based on its frequency: non-ionizing, which is characterized by low radiation (energy), such as static EMF (0 Hz), extremely low (1–300 Hz), and low-frequency EMF (300 Hz–100 kHz), radiofrequency (100 kHz–300 MHz), microwaves (300 MHz–30 GHz), and infrared; ionizing radiation, which is characterized by high radiation levels, such as ultraviolet, x-rays, and gamma-rays [14, 15, 16]. EMF can act externally and affect cells in the human body, although the cells are also characterized by their own natural endogenous, ultra-weak EMF, which is potentially generated by polar biological structures [11]. The discovery of electromagnetic radiation and the rapid improvement of technology has led to the development of medicine and new diagnostic techniques (e.g., magnetic resonance imaging) as well as therapies (e.g., tumor treating fields (TTF)) but also to improving the awareness of the possible positive and negative impacts by EMFs on cells and the human body [14]. In the context of regenerative medicine, which is a relatively new branch of medicine, most of the scientific papers that have published positive effects of EMFs have tested low-intensity EMFs, thereby showing that non-ionizing EMFs can be a useful tool in stem cell-based therapies e.g., bone and cartilage repair or wound healing [15]. EMF preconditioning (with appropriately selected parameters), which modulates the trophic activity of MSCs, may stimulate proliferation, chondrogenesis, osteogenesis, and neurogenesis, thereby enhancing the regenerative properties of those cells [10, 17].
Non-ionizing EMFs are currently being studied, and their impacts will be observed in the future. Thus, EMF can provide a very helpful, and, importantly, non-invasive biophysical tool to support stem cell-based therapy. The selection of appropriate EMF parameters, which can result in a positive biological effect that supports its therapeutic potential is crucial. In this review paper, we discuss the biological effects of extremely low-frequency electromagnetic fields (LF-EMFs) on MSCs and consider their safety and use in clinical applications.
Currently, mesenchymal stem cells are often the most considered stem cells for use in clinical applications [5]. MSCs can be found in different human tissues, such as adipose tissue (AT), bone marrow (BM), skin, dental pulp, peripheral blood, lung, heart, hair, umbilical cord, Wharton’s jelly, umbilical cord blood, and the placenta [1, 7, 18]. However, the longest utilized and most commonly considered adult tissue sources of MSCs in clinical and preclinical applications are bone marrow and adipose tissues [7, 18], which we briefly describe below.
Adipose tissue contains a heterogeneous population of cells, which only consist of approximately 20% lipid-rich adipocytes [19]. The rest of the cell population comprises stem cells, neural and vascular progenitor cells, multipotent progenitor cells, pericytes, fibroblasts and endothelial cells, eosinophils, macrophages, and innate lymphoid cells (ILCs), T cells, and B cells [7, 19]. Adipose tissues also contain adipokines, including leptin and adiponectin, which can be considered as stem cell inducers [19, 20].
There are two main types of adipose tissue, which differ in function—white (WAT) and brown (BAT) adipose tissue. WAT is responsible for the main energy storage in the organism, while BAT is an energy expenditure and protective tissue [7, 20]. Moreover, it has been shown that adipocytes from WAT can undergo differentiation in adaptive responses and reversible change functions that make them similar to BAT cells (beige AT), thereby demonstrating the plasticity of adipose tissue [20]. WAT is localized subcutaneously, intra-abdominally, epicardially, and gonadally, while BAT is interscapular, paravertebral, perirenal, cervical, and supraclavicular [21]. However, due to the ease and non-invasive harvesting procedure by liposuction, subcutaneous AT is most widely considered as a source of adipose-derived mesenchymal stem cells rather than its other localizations in the human body [7]. Importantly, uncultured stromal vascular fractions (SVFs) from adipose tissues can usually obtain up to 3% of the ASCs. However, it has been shown that SVFs from subcutaneous liposuction aspirates can contain even 10% of the ASCs [19, 22].
In clinical use, the type of adipose tissue, its harvesting area, tissue origin, age, weight, disease state, race and ethnicity, and body mass index of the donor are important factors in providing high-quality ASCs [7, 22]. It has previously been shown that ASC proliferation, differentiation potential, and growth factor secretion from older donors are lower [23, 24, 25, 26]. Moreover, the more the patient weighs may also affect lower self-renewal and angiogenic capacity but can also provide a higher adipogenic potential in isolated ASCs [7, 27, 28]. It has been also shown that smoking, diabetes mellitus, hypercholesterolemia, and hypertension have negative effects on the regenerative potential of ASCs [27, 29].
Bone marrow is a source tissue from which mesenchymal stem cells are isolated, as defined for the first time by Friedenstein et al. [6]. Although it is commonly used for research in regenerative medicine, BM as a source of MSCs also has its limitations. Obtaining tissue to isolate MSCs is an invasive procedure that can cause the patient pain and infection [24, 30].
Bone marrow, as a site for hematopoiesis, contains hematopoietic (blood cells lineages committed progenitors, stem cells) and non-hematopoietic cells (nervous system cells, adipocytes, fibroblasts, osteoblasts, osteocytes, osteoblastic precursors, mesenchymal stem cells) [6, 31]. There are two types of BM that differ in function—red (where hematopoiesis occurs) and yellow (fat storage) [32].
It is worth noting that bone marrow is a less effective source of MSCs than adipose tissue. While MSCs form 3% of adipose tissue, their content is even lower in BM (0.002%) [19]. Moreover, BM-MSCs are characterized by faster senescence and lower proliferation capacities than ASCs during expansion, and it is very important to provide medical products of high quality as fast as possible to treat the patient [1]. Adipose tissue is also a better candidate for use in regenerative medicine due to its similar properties for differentiation as BM-MSCs, yet has an easier obtaining procedure and culture expansion. However, some studies have reported better osteogenic potential in BM-MSCs than ASCs, although those studies were performed on MSCs acquired from different donors. Additionally, the MSC origin may be crucial and adaptation of the source tissue for medical purposes may depend on the disease [1, 24].
Low-frequency electromagnetic fields have been shown to influence the biological processes of mesenchymal stem cells by incorporating differentiation, proliferation, trophic activity, cell division, and metabolism, and thus, stem cell fate [10, 11, 12, 13]. Since EMFs are one of the factors that impact stem cell biology, it is very important to understand the mechanism of action on MSCs by EMFs to anticipate both the positive therapeutic effects and possible negative side effects [10]. Below, we discuss the influence of EMFs on the proliferation and cell cycle, trophic factors secretion, metabolism, and ions flow of MSCs. To our knowledge, this review and data collection on this subject are the first to be reported in the literature. Extensive discussion on the effects of EMFs and the mechanisms on the differentiations of MSCs was deliberately omitted from this review as there are many up-to-date, well-written scientific papers that have already covered the subject [9, 10, 12, 33].
Many studies have shown that appropriate EMF stimulation affects the proliferative potential and cell cycle of MSCs; however, various different effects have been observed, which suggests that the effects are dependent on the EMF parameters and stimulation times [12, 13, 34, 35, 36, 37, 38, 39, 40, 41, 42, 43, 44, 45, 46, 47].
A frequency of 50 Hz is a recognized parameter of electromagnetic fields emitted by everyday devices and has aroused the interest of researchers [34]. Li et al. [35] showed that pulsed electromagnetic fields (PEMF) (50 Hz; 10 mT) stimulation can increase the proliferation of rat MSCs after 3 and 6 h of exposure but also influence the cell cycle by increasing the percentage of cells in the G1 phase. Importantly, in that study, EMF exposure did not influence stem cell viability. Results by Li et al. [35] were a one-time effect, since, after 16 h of EMF exposure, no effect was again observed on MSC proliferation and cell cycle. In other studies, MSCs were repeatedly exposed to EMFs during the culture time with different outcomes. Fan et al. [36] and Seo et al. [37] showed that MSC proliferation increased after EMF exposure (50 Hz; 1 mT). Conversely, in work conducted by Seo et al. [37], the observed changes in proliferation were not statistically significant. Interestingly, Fan et al. [36] showed smaller changes in G2-phase cell percentage, contrary to Li et al. [35]. However, in both works, the authors indicated changes in the percentage of MSCs in S-phase post-exposure; nevertheless, as noted by Li et al. [35], this effect appeared detectable and significant 1-hour post-EMF exposure but not after 16 h [36]. This observation suggests that MSCs undergo adaptive responses to environmental conditions, such as EMFs, thereby indicating that stem cell fate can be modulated.
Alongside the 50 Hz frequency, scientists have also often studied the effects of EMFs with a frequency of 15 Hz. The results published in various research papers showed that 15 Hz can increase proliferation. Data published by Jazayeri et al. [38] showed that proliferation, after EMF exposure (15 Hz; 0.2 mT), increased, and the longer the culture underwent EMF stimulation the higher the effect. It has been shown that seeding density does not influence proliferation changes by EMF (15 Hz) stimulation; however, Sun et al. [39] showed that the slight observed increase reflected the entry of more bone marrow MSCs into the G2/M-phase at the beginning of the experiment. The number of cells at the G2/M-phase increased at the beginning and decreased after 18 h along with the number of MSCs in the S-phase, although S-phase was not affected at the beginning. Sun et al. [39] also showed that after 18 and 24 h of EMF exposure, a statistically significant higher percentage of MSCs entered into G0/G1-phase, although this effect was not observed after 30 h of EMF exposure. Song et al. [40] also discovered that the proliferation of MSCs after EMF stimulation (15 Hz; 1 mT) increased significantly after 7 and 10 days, however, this effect did not last until day 14. Similarly, using the same EMF parameters (15 Hz; 1.5 mT), other studies have also shown increased proliferation after 7 days of EMF stimulation [12, 41, 42].
Other frequencies of EMF than 15 Hz and 50 Hz were also studied. Ross et al. [43] showed a slight increase in BM-MSC proliferation using EMFs (5 Hz; 0.4 mT) for 10 min per day. However, an improved biological effect was achieved by Bloise et al. [44] (75 Hz; 2 mT, 20 min/day). A slight increase in proliferation was also shown by Poh et al. [45] (26 Hz). Thus, several studies showed EMF can increase MSC proliferation, yet Zhang et al. [13] showed no significant changes in proliferation after EMF stimulation (7.5, 15, 30, 50, 75 Hz; 1 mT) alongside results presented by Parate et al. [46] (15 Hz; 2 mT). Moreover, Yan et al. [47] indicated that EMF treatment (50 Hz; 20 mT) can inhibit MSC proliferation. The different studies on the effects of the influence of EMFs on MSC proliferation are presented in Table 1 (Ref. [12, 13, 34, 35, 36, 37, 38, 39, 40, 41, 42, 43, 44, 45, 46, 47, 48]).
Biological model | EMF parameters | Exposure duration | Biological effect | Study reference |
Rat MSCs | PEMF (50 Hz; 10 mT) | 3 h and 6 h | Time-independent increase in proliferation. Increased percentage of cells in G1 phase. | [35] |
Rat BM-MSCs | EMF (50 Hz; 1 mT) | 4 h/day for 3 days | Increased proliferation. Increased percentage of cells in S-phase. | [36] |
Rat BM-MSCs | EMF (50 Hz; 1 mT) | 1 h/day for 5, 7 and 10 days | Slight but not statistically significant increase in proliferation. | [37] |
Human BM-MSCs | EMF (7.5, 15, 30, 50, 75 Hz; 1 mT) | 24 h | No effect on MSCs proliferation for any of the tested frequencies. | [13] |
Human ASCs | PEMF (26 Hz) | 3, 7, and 14 days | Slight but not statistically significant increase in proliferation. | [45] |
Human BM-MSCs | PEMF (15 Hz; 2 mT, 3 mT) | 30 min | No significant changes in proliferation. | [46] |
Human BM-MSCs | EMF (50 Hz; 20 mT) | 12 h/day | Inhibited proliferation. | [47] |
Rat BM-MSCs | EMF (15 Hz; 0.2 mT) | 6 h/day for 5, 10, and 14 days | Increase in MSC proliferation. | [38] |
Human BM-MSCs | EMF (15 Hz) | 8 h/day for 10 days | Slight increase in MSC proliferation. Significant, time-dependent changes in G2/M- and S-phases. | [39] |
Rat BM-MSCs | EMF (15 Hz; 1 mT) | 1 h/day for 14 days | Increased proliferation. | [40] |
Rat BM-MSCs | EMF (15 Hz; 1 mT) | 4 h/day for 7 days | Increase in MSC proliferation. | [12] |
Rat BM-MSCs | EMF (15 Hz; 1mT) | 4 h/day for 7 days | Increase in MSC proliferation. | [41] |
Human ASCs | PEMF (15 Hz; 1 mT) | 8 h/day for 7, 14, and 21 days | Increase in proliferation while culturing cells on self-assembled peptide hydrogel with and without nanoparticles. | [42] |
Human BM-MSCs | EMF (5 Hz; 0.4 mT) | 10 min/day for 15 days | Slight but not statistically significant increase in proliferation. | [43] |
Human BM-MSCs | EMF (75 Hz; 2 mT) | 20 min/day, 3 times per week for 14 days | Increase in MSC proliferation. | [44] |
Human ASCs | EMF (0 Hz; 0.5 mT) | Continuous exposure for 7 days | Increase in MSC proliferation. | [34] |
Human PDLSCs | EMF (50 Hz; 1 mT) | 6 h/day for 28 days and 10 days | Increase in MSC proliferation. | [48] |
EMF, electromagnetic field; MSCs, mesenchymal stem cells; BM-MSCs, bone marrow mesenchymal stem cells; ASC, adipose-derived mesenchymal stem cells; PEMF, pulsed electromagnetic fields.
The mechanisms involved in EMFs (with different parameters) influencing MSCs
remain unclear owing to a lack of exploration. However, it is known that the
MEK/ERK signaling pathway is involved in cell proliferation, while it has been
shown that EMFs (15 Hz; 1 mT) stimulate proliferation through this pathway [40].
Similarly, EMFs (26 Hz) can increase the number of phosphorylated ERK 1/2
proteins suggesting that EMFs activate the MEK/ERK pathway in MSCs [45]. EMFs can
induce cellular stress and promote increased reactive oxygen species (ROS)
generation, which can become free radical ions. ROS are highly reactive signaling
factors that are naturally produced by cells, and depending on concentration may
have different effects on cells, including inhibiting proliferation [11].
Moreover, ion influx changes (K
Overall, EMFs can have different effects on the proliferation of MSCs depending on the parameters and duration of stimulation. EMFs have the capacity to enhance proliferation but only at specific parameters, which can be a useful tool in stem cell-based therapy, to accelerate the regeneration process via an increased number of cells capable of differentiation as well as cytokine and growth factor secretions.
MSCs offer great potential for use in regenerative medicine because of their unique secretome. In particular, ASCs deserve special attention here in relation to cell therapy owing to the extensive number of factors that they secrete, especially cell-free therapy. ASCs secrete cytokines, proteins, growth factors, and non-coding RNAs, which are carried by exosomes, and all offer great therapeutic potential. Most importantly, it was shown that EMFs with appropriate parameters can modulate the secretion of these compounds [7, 53].
EMFs can enhance regenerative properties by modulating specific signaling
pathways, gene expression, and protein secretion (Table 2, Ref.
[17, 36, 44, 46, 54, 55, 56, 57, 58]). Immunomodulatory properties can be also mediated by EMFs
[36, 46, 54]. Fan et al. [36] showed increased expression of cytokines,
such as IL-11, IL-7, LIF, SCF, M-CSF, and TPO by BM-MSCs after EMF (50 Hz; 1 mT)
stimulation but no influence in IL-6, IFN-
Biological model | EMF parameters | Exposure duration | Biological effect | Study reference |
Rat BM-MSCs | EMF (50 Hz; 1 mT) | 4 h/day for 3 days | Increase in IL-11, IL-7, LIF, SCF, M-CSF, and TPO expression. No influence on IL-6, IFN- |
[36] |
Mice BM-MSCs | PEMF (50 Hz; 1 mT) | 3 h/day | Significant increase in BDNF and VEGF expression through Wnt/ |
[55] |
Mice BM-MSCs transplanted into spinal cord injury mice model | PEMF (50 Hz; 1 mT) | 1 h/day for 8 weeks | Combined transplantation of BM-MSCs and PEMFs increases the expression of BDNF, NGF, and VEGF in vivo. | [55] |
Human BM-MSCs | PEMF (3 Hz; 80 mT, 150 mT) | 10 min/day for 14 days | Significant increase in VEGF secretion after 7 days. | [56] |
Human BM-MSCs | PEMF (15 Hz; 1G) | 21 days | Significant increase in TGF-ß after 9 days. | [57] |
Human ASCs | EMF (50 Hz; 1.5 mT) | 3 days | Significant increase in FGF-2 concentration after 1 and 2 days. Significant decrease in FGF-2 after 3 days. Slight increase in SCF, VEGF-D, VEGF-A, BDNF, and PDGF-BB after 48 h. | [17] |
Human BM-MSCs | PEMF (15 Hz; 3 mT) | 10 min | Significant increase in BMP-2, BMP-4, TSP-2, IL-1Ra, and IL-10 secretion. Slight increase in TGF-ß1, TGF-ß3, IGF-2. | [46] |
Human BM-MSCs | EMF (75 Hz; 2 mT) | 20 min/day, 3 times per week for 14 days | Slight increase in selected osteogenic proteins in proliferative medium (ALP, COL, FN, OPN, OSC, and OSN). | [44] |
Equine ASCs | EMF (0 Hz; 0.5 mT) | 7 days | Increase in BMP-2 and VEGF and decrease in TNF- |
[58] |
Human BM-MSCs | EMF (5.1 Hz; 0.4 mT) | 5 min | Decrease in IL-1b, IL-6, IL-17A, and TNF- |
[54] |
IL, Interleukin; LIF, leukemia inhibitory factor; SCF, stem cell factor; M-CSF, macrophage colony-stimulating factor; TPO, thrombopoietin; IFN, Interferon; TNF, tumor necrosis factor; FGF-2, basic fibroblast growth factor; VEGF, vascular endothelial growth factor; BDNF, brain-derived neurotrophic factor; NGF, nerve growth factor; FGF, fibroblast growth factor; PDGF, platelet-derived growth factor; TSP, thrombospondin; TGF, transforming growth factor; BMP, bone morphogenic protein; IGF, insulin-like growth factor; ALP, alkaline phosphatase; COL, Collagen; FN, fibronectin; OPN, osteopontin; OSC, osteocalcin; OSN, osteonectin.
EMFs can also modulate the trophic activity of MSCs. It has been shown that EMFs
increase the secretion of IGF-2 [46], VEGF [17, 46, 50, 55, 56], FGF-2, and PDGF
[17], although the efficiency was dependent on the EMF parameters being applied.
Studies also have shown that EMFs can influence TGF and BMP protein secretion,
which is linked to the capacity of EMFs to enhance MSC differentiation
[46, 57, 58]. EMFs (75 Hz; 2 mT) have been shown to slightly increase the
deposition of osteogenic proteins (ALP, COL, FN, OPN, OSC, and OSN) in the cell
matrix in a proliferative medium [44]. EMFs can also impact BDNF and NGF
secretion [17, 55]. Huang et al. [55] showed that treating BM-MSCs with
PEMFs (50 Hz, 1 mT) significantly increased BDNF and VEGF expression in
vitro via the Wnt/
The ability to enhance the anti-inflammatory properties of MSCs may represent an important new approach for the treatment of autoimmune diseases. Moreover, modulating the concentrations of cellular trophic factors may provide a crucial improvement in cell and tissue regeneration. However, as described and summarized in Table 2, the effects may vary depending on the EMF parameters and exposure time.
Mitochondria are extremely important organelles since they undertake the main processes of energy metabolism and adenosine triphosphate (ATP) production. These organelles also control various processes, such as cell signaling, ROS production, as well as calcium ion flow and concentration, thereby playing an important role in the proper course of key biological functions, such as differentiation or apoptosis. In response to internal and external signals, mitochondria initiate dynamic processes, such as mitochondrial fusion and fission, increasing mtDNA copy and respiratory enzymes level, enhancing oxygen consumption rate (OCR), and intracellular ATP levels to meet the metabolic demands of the cell [59, 60].
According to existing data, changes occurring under the influence of EMFs
suggest that MSCs are vulnerable to this biophysical factor and try to adapt to
new environmental conditions by changing their metabolism. MSCs are characterized
by low mitochondrial activity and glycolytic state due to their dormant form in
stem cell niches unless cells undergo differentiation (changing their fate),
which requires a high energy demand [9, 60, 61]. Hollenberg et al. [9]
showed that EMFs (10 Gauss for 4 days) significantly increased mitochondrial
membrane potential in BM-MSCs indicating increased oxidative phosphorylation
(OxPhos), and thus, mitochondrial activity and ATP production. Furthermore, a
study conducted by Ehnert et al. [62] showed that EMFs (16 Hz and 26 Hz)
can significantly increase mitochondrial activity in an osteoblast and ASC
co-culture after 7 and 14 days (5 times per week for 7 min each day).
Interestingly, Celik et al. [63] showed that the X-directed application
of PEMFs (1 mT) has a greater effect on mitochondrial respiration than Z-directed
stimulation. EMFs can influence the influx of ions, and thus, the mitochondrial
membrane potential [9, 33]. Since mitochondria store Ca
EMFs can both influence mitochondrial activity by switching glycolysis to OxPhos and increase mitochondrial DNA (mtDNA) copy numbers. Bai et al. [65]showed that EMF (50 Hz; 5 mT) increased the mtDNA copy number of BM-MSCs in a time-dependent manner, which may suggest that cells prepare for an increase in mitochondrial activity and higher cell energy demand needed for stem cell differentiation or division. It is worth mentioning that EMFs might influence mitochondrial migration, and the results we obtained showed that cells stimulated with EMFs (50 Hz; 1.5 mT) had mitochondria located closer to the periphery of the cell [data in press]. This finding may be important in understanding the regenerative potential of EMF-stimulated MSCs via mitochondrial transfer to other cells [60] since there are not many studies investigating mitochondrial transfer in physiological conditions [66].
Studies have shown that EMFs can influence mitochondria functioning; however,
the clear mechanism of action remains unknown (Table 3, Ref. [9, 62, 63, 65]). It is
suggested that EMFs induce mitochondrial ROS production on a non-toxic level
(activating TRPC1-mediated calcium entry) as well as interfere in Ca
Biological model | EMF parameters | Exposure duration | Biological effect | Study reference |
Human BM-MSCs | EMF (10 G) | 4 days | Increased mitochondrial activity. | [9] |
Rat BM-MSCs | EMF (50 Hz; 5 mT) | 30 min, 1 h and 6 h/per day for 2 days | Increased mitochondrial DNA content. | [65] |
Co-culture of human ASCs and osteoblasts | EMF (16 Hz and 26 Hz) | 14 days (5 times per week for 7 min each day) | Increased mitochondrial activity. | [62] |
Human BM-MSCs | PEMFs administrated at Z and X direction (1 mT) | 10 min | Increased mitochondrial activity, greater for X-directed stimulation. | [63] |
Electromagnetic fields can affect the ion influx of MSCs by inducing the
vibration of free ions and disturbing the electrochemical potential on the cell
membrane surface. This process may in turn affect the transmembrane receptors,
phospholipids, and in effect specific pathways that modulate stem cell
proliferation, differentiation, viability, metabolism, apoptosis, cell–cell
communication, and signaling with extracellular matrix components that influence
the stem cell fate [33, 67]. Below, we present studies (Table 4, Ref.
[13, 44, 47, 68, 69, 70, 71]) and briefly describe the influence of EMFs on K
Biological model | EMF parameters | Exposure duration | Biological effect | Study reference |
Human BM-MSCs | PEMF (75 Hz; 2 mT) | 10 min/day | Increased Ca |
[68] |
Human BM-MSCs | PEMF (50 Hz; 1 mT) | 24 h | Increased concentration of intracellular Ca |
[13] |
Human BM-MSCs | EMF (50 Hz; 20 mT) | 12 h/day | Increased concentrations of K |
[47] |
Human BM-MSCs | EMF (75 Hz; 2 mT) | 20 min/day, 3 times per week for 14 days | Increased concentration of intracellular Ca |
[44] |
Human BM-MSCs | EMF (45 Hz; 1 mT) | Twice every 8 h/day for 7 days | Significantly increased expression of L-type VGCCs CACNA1E and CACNA1G but only slight increase of CACNA1C and CACNA1I. | [69] |
Human BM-MSCs | PEMF (15 Hz; 2 mT) | 10 min | Increased expression of TRPC1 and TRPV4 genes related to channels for calcium entry. No significant influence on L-type VGCC gene expressions (CACNA1C and CACNA2D1). | [70] |
Human BM-MSCs | EMFs (7.5, 15, 30, 50, and 75 Hz; 1 mT) | 8 h/day | Increased purinergic receptor P2X7 expression. | [71] |
VGCCs, voltage-gated calcium channels; FAK/Rho GTPased, focal adhesion kinase/ras homologous guanosine triphosphatase.
Yan et al. [47] showed increased K
EMFs can also increase calcium currents [72] and the intracellular concentration
of calcium ions in MSCs [13, 44, 47, 68] by activating the L-type voltage-gated
calcium channels (VGCCs) through membrane depolarization [68, 69] and
significantly increasing the expression of VGCC-related genes (CACNA1E and
CACNA1G) [67, 69]. Interestingly, two independent studies showed that EMF exposure
at different parameters did not influence CACNA1C gene expression related to the
VGCC [69, 70]. Moreover, it has been shown that EMFs increased the expression of
the TRPC1 and TRPV4 genes involved in the entry of calcium through the cell
membrane [70] as well as P2X7 purinergic receptors related to the transport of
sodium, calcium, and potassium [71]. Influencing calcium channels may have an
effect on the differentiation and migration of MSCs, while Zhang et al.
[13] showed that EMF-mediated changes in Ca
Electromagnetic fields can influence ion influx, and thus, regulate stem cell functioning, which may be useful in the context of using MSCs as therapeutic treatments. Calcium has the potential to regulate stem cell fate mediated by EMF, while the disturbance of this concentration may lead to both desired and undesirable effects, such as faster differentiation, increased proliferation, or cell death. More research is needed on the effects of EMFs to explore the impact of EMFs on the flow of ions in MSCs, especially from sources other than BM-MSCs.
Many in vitro and in vivo studies have demonstrated that
electromagnetic fields can also influence the differentiation of mesenchymal stem
cells [9, 10, 12, 33]. EMFs influence the influx of ions, and thus, the
concentration across the cell membrane and transmembrane potential. In turn, this
influences cell–cell communication and cell physiological processes, thereby
modulating stem cell fate by triggering epigenetic changes and gene expression
towards the activation of differentiation pathways [10, 11, 33, 74]. Electromagnetic
fields, as suggested by Bai et al. [65], may cause the MSCs to become
more sensitive to environmental changes, which leads to easier differentiation.
In fact, EMFs influence Ca
Clinically, most interest is focused on the chondrogenesis and osteogenesis of MSCs, while neurogenesis has also been widely studied. Previously, Safavi et al. [10] published a systematic review covering the topic of MSC differentiation based on literature published up to December 2021. In this review paper, we only collected research papers available in PubMed that studied MSC chondrogenic, osteogenic, and neural differentiations published between January 2022 and July 2023 (Table 5, Ref. [48, 55, 56, 75, 76, 77, 78, 79, 80, 81]).
Biological model | EMF parameters | Exposure duration | Biological effect | Study reference |
Mice BM-MSCs | PEMF (50 Hz; 1 mT) | 1 h and 3 h/day | Improvement of neural functions and axon connection. Recovery after spinal cord injury is promoted via the Wnt/ |
[55] |
Human BM-MSCs | PEMFs (30, 45, 60, and 75 Hz; 10 mT) | 30 min/day for 3 days | Upregulation of MAP-2 and NF-L after exposure to 60 and 75 Hz. Significant increase in neural-related protein expressions. Reduction in MMP-9, TNF- |
[75] |
Human BM-MSCs | PEMF (3 Hz; 80 mT, 150 mT) | 10 min/day for 14 days | Increased expressions of COL-I, ALP, and BMP-2, although not significant. | [56] |
Rat ASCs | PEMF (50 Hz; 0.6 |
30 min/day for 3 weeks | Enhanced osteogenesis. Significant increase in calcium deposition in cells after PEMF on 1% RGO. | [76] |
Rat BM-MSCs | EMF (15 Hz; 1 mT) | 1 h/day for 21 days | Increased osteogenesis on Fe |
[77] |
Human PDLSCs | EMF (50 Hz; 1 mT) | 6 h/day for 28 days and 10 days | Statistically significant increase in calcium deposition. Upregulation of RUNX-2, COL-1A1, and OPN expression. | [48] |
Rat ASCs | EMFs (0, 15, 45, and 75 Hz; 1 mT) | 24 h | ASC-derived exosomes: suppressed inflammation and extracellular matrix degeneration; upregulated COL-2A1, Sox-9, and ACAN expressions and also improved regeneration of osteoarthritic cartilage in vivo. | [78] |
Mice BM-MSCs | EMF (0 Hz; 200 mT) | 14 days | Increased expressions of Sox-9 and COL-2. Induced MSC migration through SDF-1/CXCR4. | [79] |
Human BM-MSCs | EMF (15 Hz; 10 T/s) | 3 h/day for 14 days | Promoted Sox-9, ACAN, COL2A1, and matrix protein collagen type II expression. Inhibited expression of degeneration matrix protein collagen type X and hypertrophic genes. | [80] |
Human and rat BM-MSCs | EMF (15 Hz; 10 T/s) | 3 h/day for 21 days | Increased expression of Sox-9, ACAN, and COL2A1. Regulated chondrogenesis through ERK and p38 MAPK pathways. | [81] |
MAP, microtubule-associated protein; NF-L, neurofilament light chain; MMP, metalloproteinase; TNF, tumor necrosis factor; RGO, reduced graphene oxide; OCN, osteocalcin; RUNX2, Runt-related transcription factor; ACAN, aggrecan.
Recently, Huang et al. [55] showed that a combined treatment of EMF (50 Hz; 1 mT) and BM-MSCs may enhance and promote spinal cord injury treatment in a mice model by increasing the expression of NGF, BDNF, and VEGF. It has been also reported that EMFs can increase neuron preservation (NeuN and NF-200) and increase axonal growth (MBP, myelin sheath) [55]. Moreover, it has been shown that an approach combining EMFs and MSCs may be successful for use in cerebral ischemic models. Park et al. [75] showed that EMFs (60 and 75 Hz; 10 mT) can increase the protein expression of MAP-2 and NF-L in cell cultures of BM-MSCs, while BM-MSCs/EMF (60 Hz; 10 mT) treatment can reduce inflammation and significantly improve behavior and motor coordination in a cerebral ischemic mice model after treating for 13 days. Interestingly, Guo et al. [82] managed to provoke ASCs to undergo neuronal differentiation by using biodegradable graphene film, which acted like a wireless electrical signal generator led by electromagnetic induction.
Seonwoo et al. [76] studied the effects of applying reduced graphene
oxide-incorporated natural calcium phosphate cements (RGO-CPCs) and EMF (50 Hz;
0.6
An interesting study that used ASC-derived exosomes in osteopathist treatment,
was performed by Xu et al. [78]. They reported that EMF exposure reduced
inflammation and extracellular matrix degeneration in ASC-derived exosomes as
well as increased COL2A1, SOX9, and ACAN expression in chondrocytes. Moreover,
using the osteoarthritis rat model, authors showed that injecting EMF (75
Hz)-exposed ASC-derived exosomes promoted the regeneration of osteoarthritic
cartilage [78]. A different study, performed by Sun et al. [79], also
showed that static magnetic fields can increase COL2A1 and SOX9 expression and
improve the migration of BM-MSCs through SDF-1/CXCR4. Additionally, Zhang
et al. [13] showed that LF-EMFs can promote MSC migration by
accumulating Ca
Studies have shown that EMFs have a positive effect on MSC differentiation and can improve as well as accelerate this process. Thus, EMFs represent an easy-to-apply tool that can be considered for use in stem cell therapy.
MSCs can be obtained from most human tissues but with different efficiencies. Currently, the main sources of MSCs for consideration in stem cell-based therapy are bone marrow, adipose tissue, and the umbilical cord [1, 7, 83]. Interestingly, the differentiation of iPSCs into MSCs represents a new approach to obtaining MSCs and may be useful in autologous treatment using MSCs in geriatric patients [66]. MSCs can be considered in the treatment of various diseases and conditions in the fields of dermatology, neurology, pulmonology, cardiology, orthopedics, and immunology [7]. According to the clinical trial experiences of Hoang et al. [1], the tissue from which the MSCs originate is important for the targeted therapies and clinical applications with MSCs. Hoang et al. [1] supported the hypothesis that bone marrow can be a good source of MSCs for treating brain and spinal cord injuries, while MSCs originating from adipose tissues are good for treating reproductive disorders and skin regeneration; MSCs from umbilical cords can be used to treat pulmonary disease and acute respiratory distress syndrome.
Safety and effectiveness are mandatory criteria for introducing stem cell-based therapies as a standard but these require validated clinical trial results [2]. The clinical use of MSCs has its limitations, which are ascribed to the variable immunocompatibility, stemness stability, heterogeneity, differentiation, and migratory and homing capacity of MSCs. The unexpected behavior of MSCs in clinical settings might occur from difficulties in the production and administration of MSC-based medical products. These challenges require an appropriate approach, and for this reason, the preconditioning of cell culture through the application of biochemical or physical inducers (such as drugs or EMFs) as well as genetic modification of MSCs is being considered. Several studies that have preconditioned the MSCs are currently registered in the ClinicalTrials.gov database [33, 84, 85]. It is worth noting that EMF preconditioning can be precise and efficient (if the appropriate EMF parameter is selected) and more cost-effective during the process of in vitro cell differentiation compared to using synthetic growth factors, which are expensive and often pleiotropic. EMF preconditioning may also be more time-efficient and more personalized in the context of preparing stem cell-based medical products by increasing the rate of cell proliferation and modulating differentiation, which are important in autologous therapies.
Intense and rapid technological development has raised public concerns about the safety of EMFs on human health. At this point, it should be noted that these concerns may indeed be compounded by the increasing number of electromagnetic field sources in the environment and the statement by the International Agency for Research on Cancer (ICAR), which classified radiofrequency with the ability to induce potentially harmful effects, although low-frequency EMFs were not classified to this effect [15]. The World Health Organization (WHO) has also stated that there is currently no evidence of harmful effects being induced by low-level exposure to EMFs [86]. Moreover, the WHO is coordinating an international project to publish systematic reviews on the effects of radiofrequency EMFs on health outcomes [87]. Studies conducted in vitro showed no negative effect of LF-EMFs on the morphology or viability of MSCs [17, 35, 69], as well as on their karyotype [43]. However, the research was performed by researchers from around the world and was limited to only specific parameters of electromagnetic fields, thereby again reaffirming that the biological effect observed in vitro/in vivo is dependent upon the specific conditions of EMF exposure. Moreover, it is important to note that the MSC culture conditions are very important, while the composition of the culture medium may also influence the obtained results. It is suggested in protocols for medical products that MSCs should be cultured without fetal bovine serum (one alternative is culturing with human Platelet Lysate), although this can affect the cellular conditions and raises concerns regarding product safety and ethical problems [88, 89]. Furthermore, the use of antibiotics in cell culturing may cause undesirable effects on the MSCs [90]. Skubis et al. [90] showed that amphotericin B can decrease cell viability, while a penicillin–streptomycin mixture can affect stemness phenotype, adipogenesis, and osteogenesis of human ASCs. In addition to streptomycin, amikacin has been shown to have a negative effect on MSCs when used at high concentrations [91].
Based on the data collected above in Chapter 2, we summarized the electromagnetic field parameters used in those studies (Fig. 1), concluding that most studies used testing frequencies of 15 and 50 Hz, while the induction of the magnetic field was 1 mT. However, the parameters used in the differentiation studies presented in this paper were not included due to the fact that this article does not present a broad analysis of this area of the literature, and instead, narrowed down the publication window to papers published between January 2022 and July 2023, as explained in Section 3.5. We also prepared a schematic representation of the possible influence of EMFs on MSCs based on the studies collected (Fig. 2).
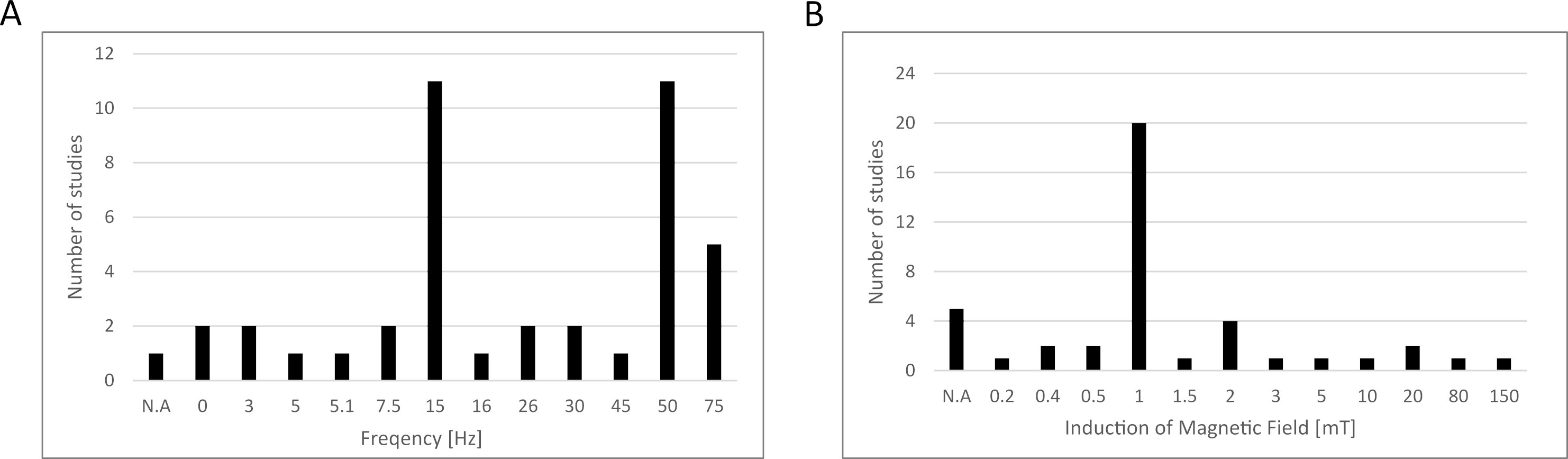
Electromagnetic field parameters used in the studies collected in this review. (A) Frequency, (B) induction of magnetic field. N.A., data not available.
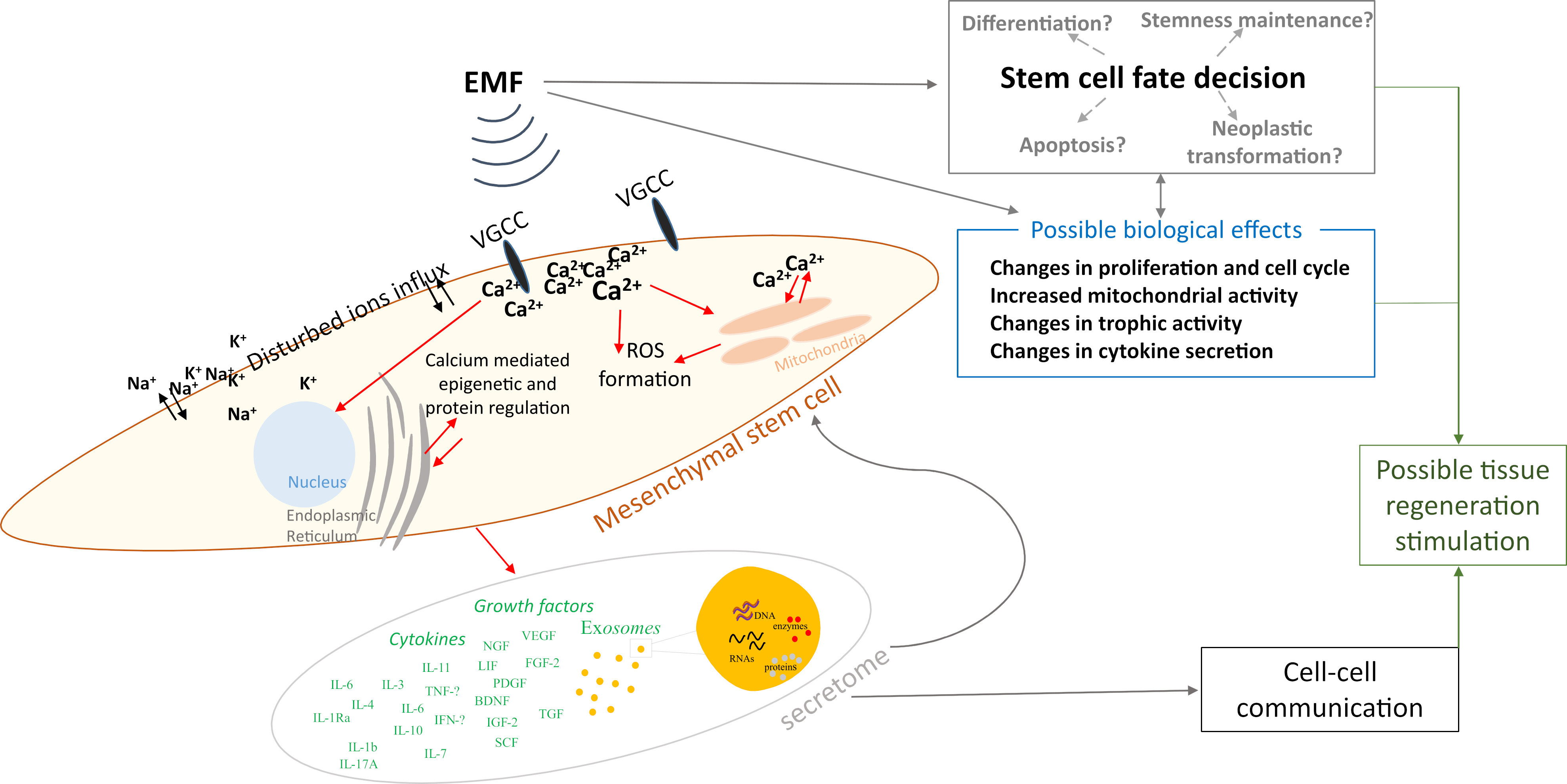
Schematic representation of the possible influence of electromagnetic fields on mesenchymal stem cells. ROS, reactive oxygen species.
Electromagnetic fields were initially approved as an effective health therapy by the Food and Drug Administration (FDA) in 1979 [56], while MSCs were first used in human subjects in 1995 [92]. Currently, two clinical trials are registered in the clinicaltrials.gov database that uses a combined therapeutic approach of EMFs and MSCs (Table 6), with both studies applying Wharton’s jelly-derived MSCs (WJ-MSC) for ophthalmic use. A phase 3, open-labeled clinical study (NCT04877067) reported that treatment with MSCs and EMFs (42 Hz; 2 G for 30 min) can be effective in toxic optic neuropathy and can prevent permanent blindness [93]. The same group also tested a similar approach for treating retinitis pigmentosa (NCT05800301) but results are not yet available. Based on this, combination therapy using EMFs and MSCs appears to be well tolerated and effective, not only in vitro and in vivo but also in human subjects.
NCT number | Study title | Condition | Interventions |
NCT04877067 | Therapy of Toxic Optic Neuropathy Via Combination of Stem Cells with Electromagnetic Stimulation (Magnovision) | Toxic optic neuropathies | Wharton’s Jelly-derived Mesenchymal Stem Cells and repetitive electromagnetic field stimulation for treating patients’ eyes. |
NCT05800301 | Management of Retinitis Pigmentosa Via Combination of Wharton’s Jelly-derived Mesenchymal Stem Cells and Magnovision | Retinitis pigmentosa | Wharton’s Jelly-derived Mesenchymal Stem Cells and repetitive electromagnetic field stimulation for treating patients’ eyes. |
The use of LF-EMFs in preconditioning MSCs can be an effective approach to
omitting the current limitations associated with the use of MSCs as a therapeutic
treatment. Moreover, adjusting the EMF exposure parameters can control the stem
cell fate and lead MSCs toward the desired therapeutic effect. LF-EMFs positively
affect the proliferation, migration, differentiation, and immunomodulatory
properties of MSCs and can be used in medicine for conditions where inflammation
and tissue damage occur—for example—in wound healing, bone and cartilage
regeneration, or regeneration after stroke. The exact mechanism through which
EMFs act on MSCs is not yet known, although it is being intensively studied. It
is currently known that EMFs affect the Ca
AS conception of the project, collection, assembling, analysis and interpreting data, designing and writing the manuscript, tables and figures preparation. BP collection and assembling data, manuscript writing. ABZ conception of the project, coordinating and revising the manuscript critically for important intellectual content, final approval of manuscript. All authors read and approved the final manuscript. All authors have participated sufficiently in the work and agreed to be accountable for all aspects of the work.
Not applicable.
Not applicable.
This research received no external funding.
The authors declare no conflict of interest. Given her role as Guest Editor, Agnieszka Banaś-Z
Publisher’s Note: IMR Press stays neutral with regard to jurisdictional claims in published maps and institutional affiliations.