- Academic Editor
†These authors contributed equally.
Background: In addition to intrahepatic angiogenesis, patients with cholestasis cirrhosis develop extrahepatic vasculature disorders and functional disturbances of multiple organ systems. Without effective intervention, these vascular disorders will eventually turn into multiple organs vascular syndromes, including the brain, lung and other organ systems. However, studies on the pathogenesis of vascular alterations among extrahepatic organ disturbances are still carried out separately, which hampered the successful translation of preclinical studies to the human setting and required further mechanistic insight into these complications. This study aims to investigate the relationship between extrahepatic angiogenesis and multiple organ impairment, and whether the vascular endothelial growth factor (VEGF) family members and their receptors are involved in this process. Methods: Pathological changes of the multiple organs were determined by histopathological and immunohistochemical staining in the established common bile duct ligation (CBDL) rats, and angiogenesis was estimated by microvessel density (MVD). Levels of the VEGF family members and their receptors in the serum and organ tissues were also measured by using enzyme-linked immunosorbent assays. Results: The MVD and VEGF family members and their receptors were significantly increased in CBDL rats with multiple organ injury, especially in the liver, lung and cerebral cortex. Meanwhile, we noticed moderate elevation of soluble receptor of the vascular endothelial growth factor-1 (sFlt-1) in the liver, lung, and cerebral cortex, whereas the levels of placental growth factor (PLGF) increased significantly. Conclusions: Extrahepatic angiogenesis may represent a common pathophysiological basis for multiple organ dysfunction and the sFlt-1/PLGF ratio could offer an avenue for further studies to target extrahepatic angiogenesis in cholestatic cirrhosis.
Cirrhosis is the final progression of liver fibrosis caused by many forms of liver diseases and conditions. While people with early compensated cirrhosis have no signs or complications of cirrhosis, serious complications including portal hypertension (PH), hepatic encephalopathy, hepatopulmonary syndrome, hepatorenal syndrome and coagulation disorders in the late-stage of cirrhosis contribute to the high mortality [1]. PH is a major complication of cirrhosis and is associated with poorer outcomes [2].
There is increasingly recognized that angiogenesis plays a substantial role in the development of PH [3]. Indeed, the newly formed blood vessels bypassed sinusoids unable to provide oxygen to tissues, which deteriorates the course of the disease and aggravates hepatic vascular resistance to portal blood flow [4, 5, 6, 7]. Further development of PH involves angiogenesis in the complex processes of restructuring the portal-systemic vascular bed [8, 9, 10, 11]. Etiologically, PH is not isolated phenomenon and hemodynamic disorders associated with PH in liver cirrhosis may contribute to the disturbances in different systems, which could finally lead to multiorgan disorders [12, 13]. In patients with advanced liver cirrhosis, increased portosystemic shunting promotes the portal venous vasoactive factors bypass the hepatic “filter” and, thus, may cause severe complications, including hepatopulmonary syndrome (HPS), hepatic encephalopathy, hepatorenal syndrome, etc. [11, 13, 14, 15, 16]. Although the pathogenesis of these complications remains poorly understood, pathological pulmonary angiogenesis has been notably recognized as one of the key pathogenic features of HPS [17, 18]. In addition, Rautou and his team [19] found that HPS was associated not only with significant intrahepatic vascular changes but also with features suggestive of angiogenesis in the right colon wall. Observational studies suggest that antiangiogenic therapies, including inhibiting extrahepatic angiogenesis, are considered as a breakthrough method of treating associated complications in animal experiments [17, 20, 21]. These findings raise an interesting new question that extrahepatic organ injuries may share a common pathophysiological mechanism, which may be particularly relevant to pathological angiogenesis.
Actually, there are many mediators involved in angiogenesis in context of liver cirrhosis. Research data refers to the complications of cirrhosis have indicated that elevated levels of circulating angiogenic factors are mainly derived from the injured liver and linked to persisting pathological angiogenesis, among which VEGF, VEGFR-2, placental growth factor (PLGF) and soluble vascular endothelial growth factor 1 (sFlt-1) all play the role of activation or inhibition [22, 23]. In animal models, many studies have shown that pulmonary angiogenesis is accompanied by the accumulation of VEGF-A in the lung, and the activation of VEGF-associated angiogenic pathways in HPS [18]. PLGF, a pleiotropic cytokine belonging to the VEGF family, has also been found to contribute to pathological angiogenesis in PH and HPS [10, 17]. Meanwhile, the VEGF family members and their receptors were also increased in serum and other tissues in rats with secondary biliary cirrhosis induced by common bile duct ligation (CBDL) [17]. As with the clinical findings, CBDL rats were also hyperdynamic, and in addition, with systemically pathological angiogenesis demonstrated by increased microvessel density (MVD) in the mesenteric window [19, 21]. A potential role for therapies that targeting VEGF pathway has been shown to be effective in suppressing extrahepatic angiogenesis, reducing portal pressure and its complications, reducing portal-systemic collateral shunting [24]. These findings of directly targeting proangiogenic pathway for intra- and extrahepatic angiogenesis implied the fundamental pathophysiological mechanisms in liver cirrhosis.
Dissatisfaction with the current methods of pharmacotherapy, as well as progress in understanding the pathogenesis of liver cirrhosis, make finding pathophysiological mechanisms for the prevention and treatment of its complications a vital task. Therefore, we aimed to investigate whether pathological angiogenesis and VEGF family/receptor changes are one of the common pathophysiological mechanisms of extrahepatic multiple organ dysfunction in cholestatic cirrhosis.
Male Sprague–Dawley rats (200~220 g, Animal laboratory center
of Third Military Medical University) were housed in a complex housing system
consisting of standard cages in a temperature-controlled room at 22
At the end of the experiment, rats were sacrificed after anesthetized with 3%
pentobarbital sodium (30 mg/kg, intraperitoneal injection) and arterial blood
from abdominal aortic was collected. Part of the arterial blood was sent for
arterial blood gas analysis by a standard blood gas analyzer (Radiometer ABL800
FLEX, Copenhagen, Denmark) within 15 minutes, liver function test using the
instruments of Automatic Biochemistry Analyzer (AU5400, Olympus, Tokyo, Japan),
and kidney function test separately. The alveolar-arterial oxygen gradient
(P
Immediately after surgical removal, the livers, lungs, kidneys, spleens, intestines, and brains were fixed in 10% formalin for 24 h. Rats tissues were then dehydrated, embedded in paraffin, and cut into 4-µm-thick slices. Tissue sections were stained with hematoxylin and eosin (the lung, kidney, spleen, intestines, and brain) and sirius red (liver) to detect morphological changes as previously described [17, 21, 25]. The microphotographs of the specimens were obtained with a light microscope (OlympusBX51-PMS, Tokyo, Japan). The degree of extrahepatic organ injury and liver fibrosis were evaluated on the HE-stained tissues sections and METAVIR score on sirius red-stained liver sections. Five randomly selected fields of each section from three different rats (the selected samples are very typical and representative) in each group were analyzed in a blinded manner.
Injury of organs (the liver, lung, kidney, spleen, intestine, and brain) are
classified according to pathologic criteria as follows: (A) METAVIR score of
liver fibrosis (F0: no fibrosis; F1: Portal fibrosis without septa; F2: Portal
fibrosis with rare septa; F3: Numerous septa without cirrhosis; F4: Cirrhosis)
[27]. (B) Lung injury score (grade 0: normal appearance, negligible damage; grade
1: mild-moderate interstitial congestion and neutrophils in the interstitial
space; grade 2: perivascular edema formation, partial destruction of lung
architecture and moderate cell infiltration; grade 3: moderate lung alveolar
damage and intensive cell infiltration; grade 4: severe cell infiltration and
destruction of the lung architecture.) [28]. (C) Kidney index (kidney indexes =
[kidney weight (g)/body weight (g)]
The assessment of angiogenesis in various tissues (the liver, lung, kidney,
spleen, intestine, and cerebral cortex) was conducted on three rats in each
group. Paraffin-embedded above organs (4 µm) were dewaxed and hydrated;
antigen was repaired by EDTA. After 10% bovine serum albumin was blocked for 1 h
at room temperature, the sections were incubated by anti-CD31 (ab119339, 1:100,
Abcam, Boston, MA, USA) antibody overnight. The next day, after washing with PBS,
sections were incubated with Cy3-conjugated Goat Anti-Mouse IgG (H+L) (115165003,
1:500, Jackson, West Grove, PA, USA), an anti-mouse secondary antibody for 1 h at
room temperature. After washing with PBS three times, sections were fixed with
4
The liver and renal function were determined by the level of alanine transaminase (ALT), aspartate transaminase (AST), urea nitrogen (Urea), creatinine (Crea), cystatin C (Cys-c) in the rat serum by Automatic Biochemistry Analyzer (AU5400, Olympus, Tokyo, Japan).
To explore the possible pathophysiological mechanism of angiogenesis in different organs, the Elisa assay was used to determine the angiogenesis-associated factors in rats, including VEGF, VEGFR1, VEGFR2, soluble vascular endothelial growth factor 1 (sFlt-1) and PLGF. Elisa kits for rats were VEGF (JL21369), VEGFR1(JL21373), VEGFR2 (JL21374), PLGF (JL11559), and sFlt-1 (JL48077), respectively. All the Elisa kits were purchased from Shanghai Jianglai Biological Technology, Shanghai, China. All the procedures were strictly following the instructions of manufacturer. The intensity of the color was measured at the absorbance of 450 nm with a Thermo Reader (Varioskan Flash Multimode Reader, Thermo scientific, Waltham, MA, USA). Serum values were expressed as pg/mL. Various tissues values were expressed as pg/mg.pro (the amount of target protein per unit protein).
Descriptive statistics are summarized as the mean
CBDL is a typical model of cholestatic cirrhosis and is often used to study
cholestatic cirrhosis and secondary extrahepatic organ damage [25, 29].
Compared with the sham group, liver exhibited cirrhosis features with
significantly elevated AST and ALT levels (Fig. 1a,c,e,k,h). Meanwhile, lung
injury was evidenced by the histopathological features of pulmonary vascular
proliferation and decreased P
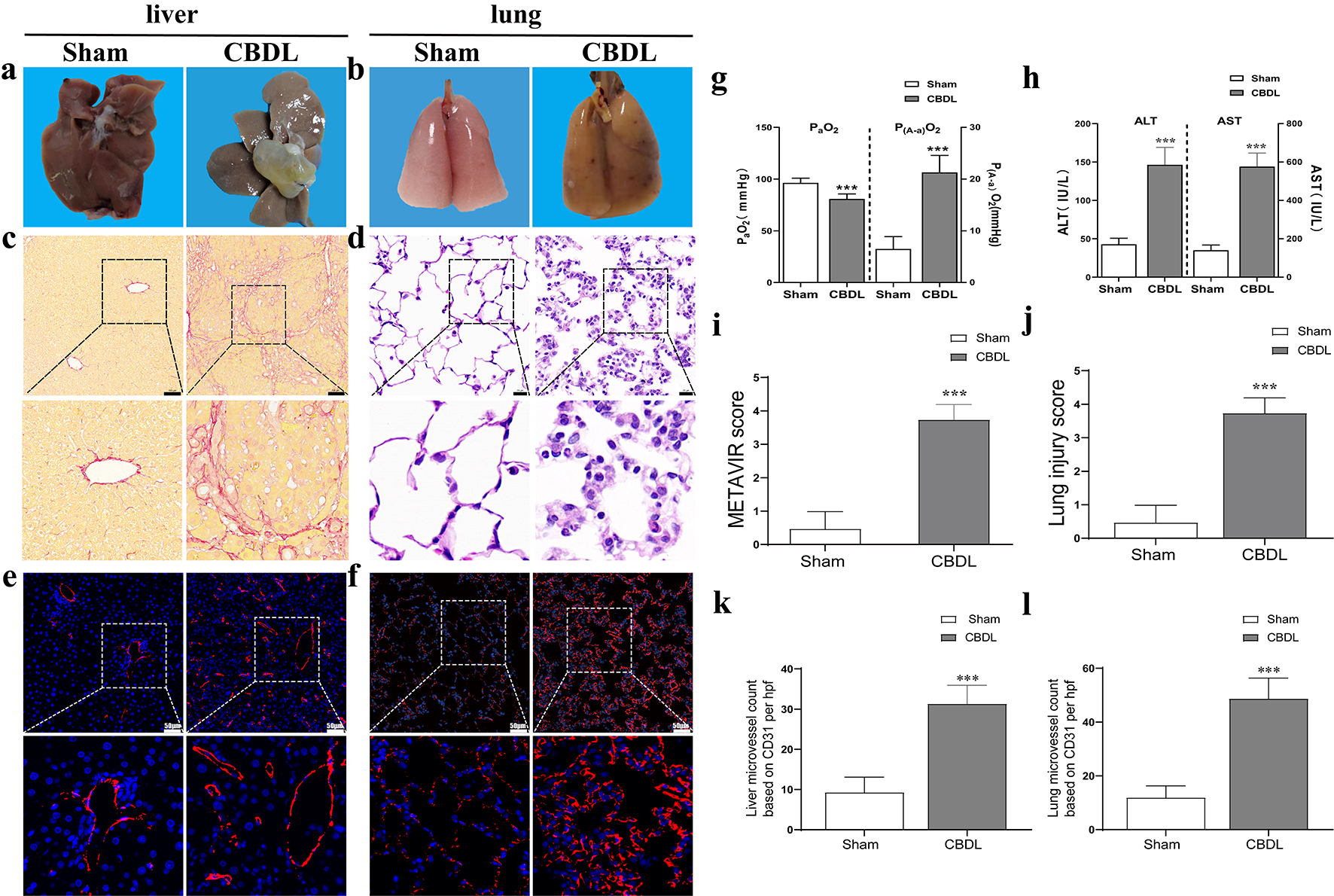
Successful establishment of CBDL rat model. (a) The
morphological and pathological changes of the liver after CBDL. (b) The
morphological and pathological changes of the lung after CBDL. (c) The
pathological changes of the liver after CBDL (scale bar = 100 µm) and (i)
liver injury score (assessed by METAVIR score). (d) The pathological changes of
the lung (scale bar = 20 µm) and (j) injury score (assessed by lung injury
score) after CBDL. The microvessel density in CBDL rats’ liver (e,k) and lung
(f,l), the slice field of view is 200 times, with a ruler length of 50 µm.
(g) Impairment of oxygenation in HPS rats. (h) Liver injury evidenced by liver
function test in HPS rats. Data were represented as mean
In addition to the aforementioned injuries of the liver and lung injury (Fig. 1i,j), damage to the kidney, spleen, small intestine and cerebral cortex was also examined. Renal injury was confirmed by increased renal index and elevated biochemical markers (e.g., blood urea, serum creatinine, and Cys-C) in the CBDL group, as well as histopathological features of biliary pigment deposition and tubular dilation (Fig. 2d,h,j,k,l). Spleen injury was also manifested by the increased volume, fibrous thickening of the splenic capsule and spleen index (Fig. 2b,f); small intestines injury was evidenced by edema, the increased villi width and number of internal red blood cells (Fig. 2c,g,i); and brain injury reduced normal neurons (Fig. 2a,e) in CBDL rats.
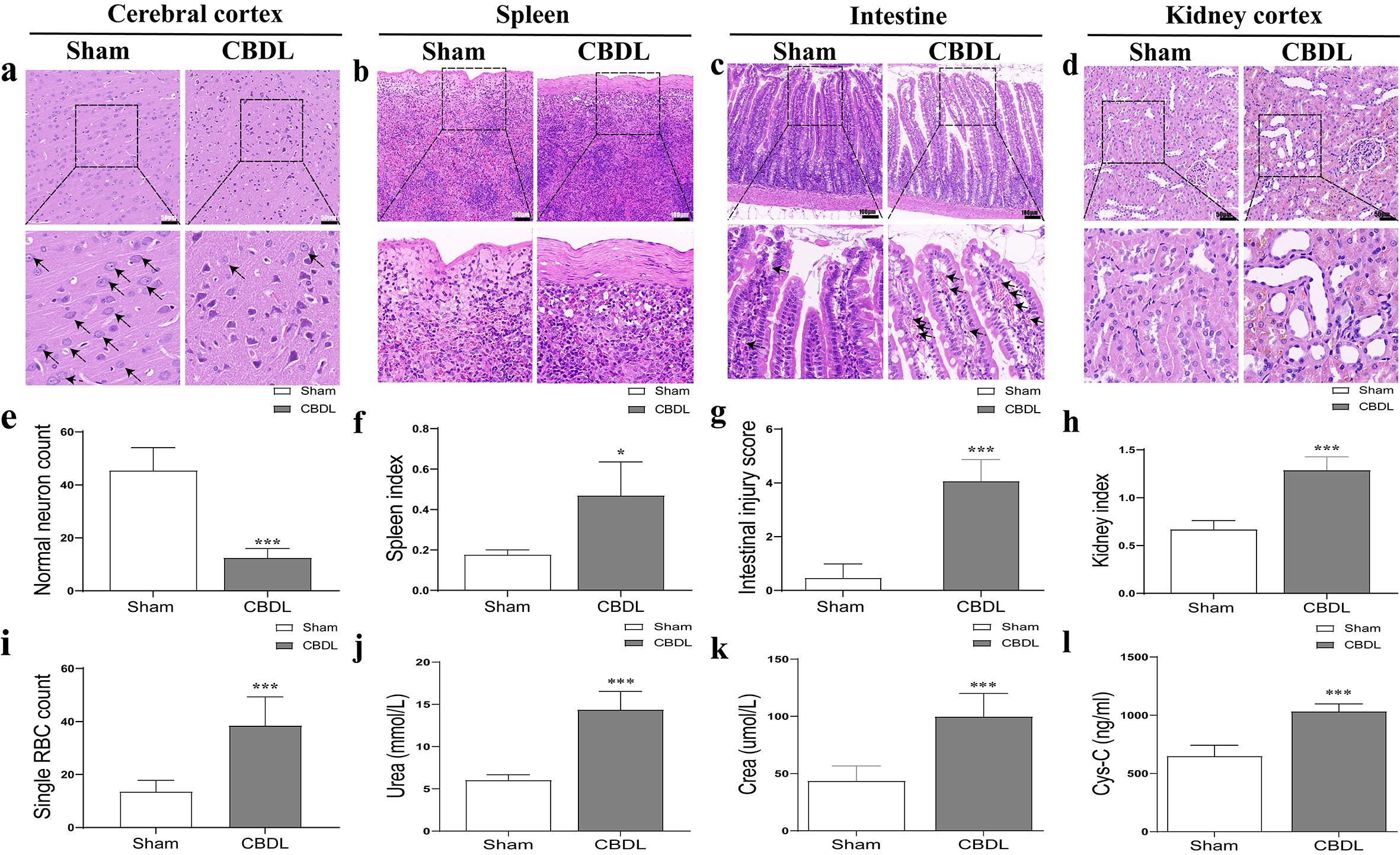
Pathological changes of extrahepatic organs in CBDL
rats. (a) The pathological changes of the cerebral cortex (scale bar = 50
µm) and (e) normal neuron count (based on HE) after CBDL. (b) The
pathological changes of the spleen (scale bar = 100 µm) and (f) spleen
index after CBDL. (c) The pathological changes of the intestine (scale bar = 100
µm), (g) intestinal injury score and (i) single RBC count (based on HE)
after CBDL. (d) The pathological changes of the kidney (scale bar = 50 µm)
and (h) kidney index (based on HE) after CBDL. (j) The plasma urea nitrogen
(Urea) changes after CBDL. (k) The plasma creatinine changes after CBDL. (l) The
plasma cystatin C changes after CBDL. Data were represented as mean
We furtherly found that the MVD of the liver (Fig. 1e,k), lung (Fig. 1f,i), cerebral cortex (Fig. 3a,e), intestine (Fig. 3c,g), kidney (Fig. 3d,h), but not the spleen (Fig. 3b,f), was significantly increased (2–4 times) in CBDL rats, with the highest increase of MVD in the liver, lung, and cerebral cortex accompanied by more severe injury (Fig. 1 and Fig. 3). Meanwhile, the expression of VEGF, VEGFR2, PLGF, and sFlt-1 in the corresponding MVD-increased organs of CBDL rats was also significantly elevated (Figs. 4,5). Our results suggest that extrahepatic injury and pathological angiogenesis were common in CBDL rats, accompanied by significant changes in the VEGF family and its receptors, specific for tissue and angiogenesis-associated factors.
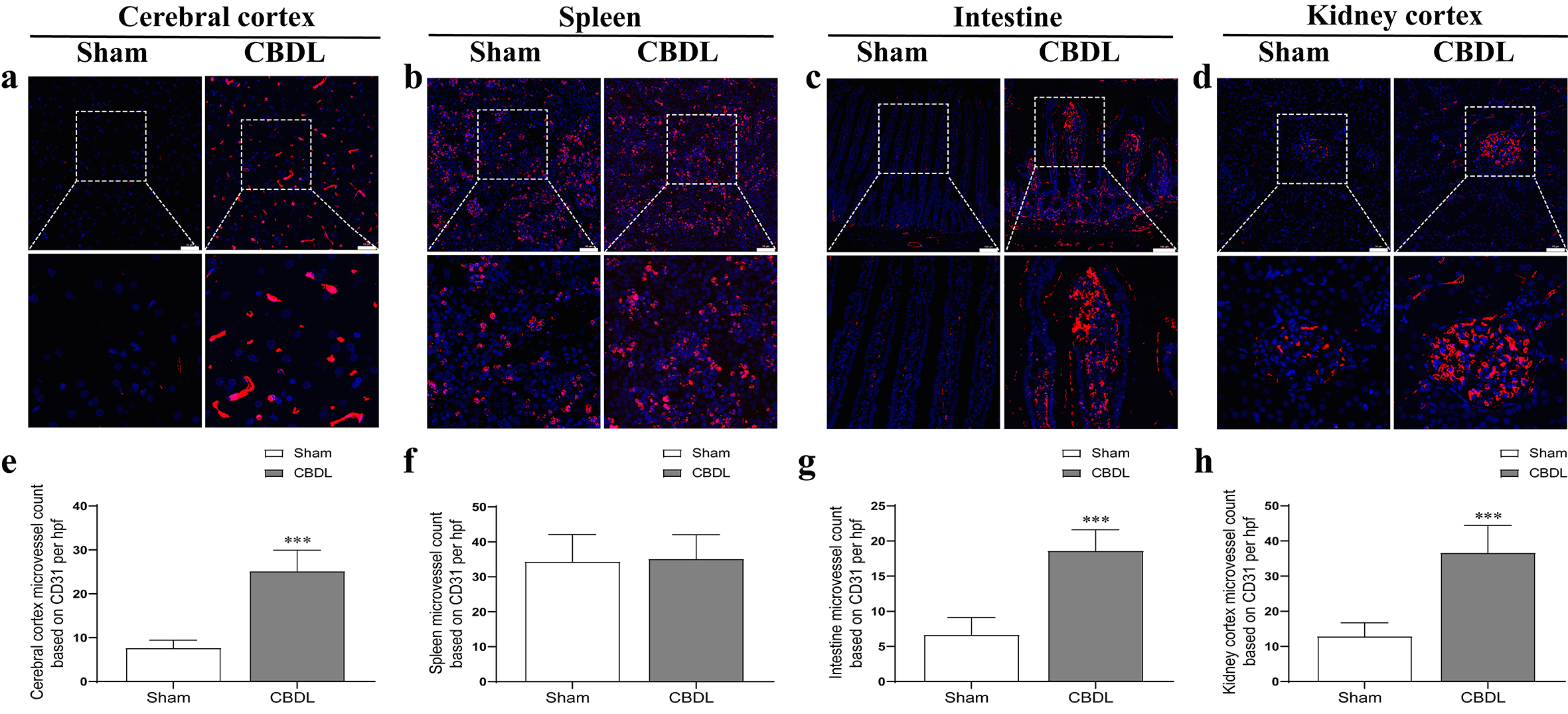
The microvessel density changes of extrahepatic organs
in CBDL rats. The microvessel density changes in cerebral cortex (a), spleen
(b), intestine (c), kidney cortex (d) after CBDL. The graphical summary of
microvessel density changes in cerebral cortex (e), spleen (f), intestine (g),
kidney cortex (h) after CBDL. The slice field of view is 200 times of the kidney,
spleen, and cerebral cortex, with a ruler length of 50 µm; small intestine
100 times, with a ruler length of 100 µm. Data were represented as mean
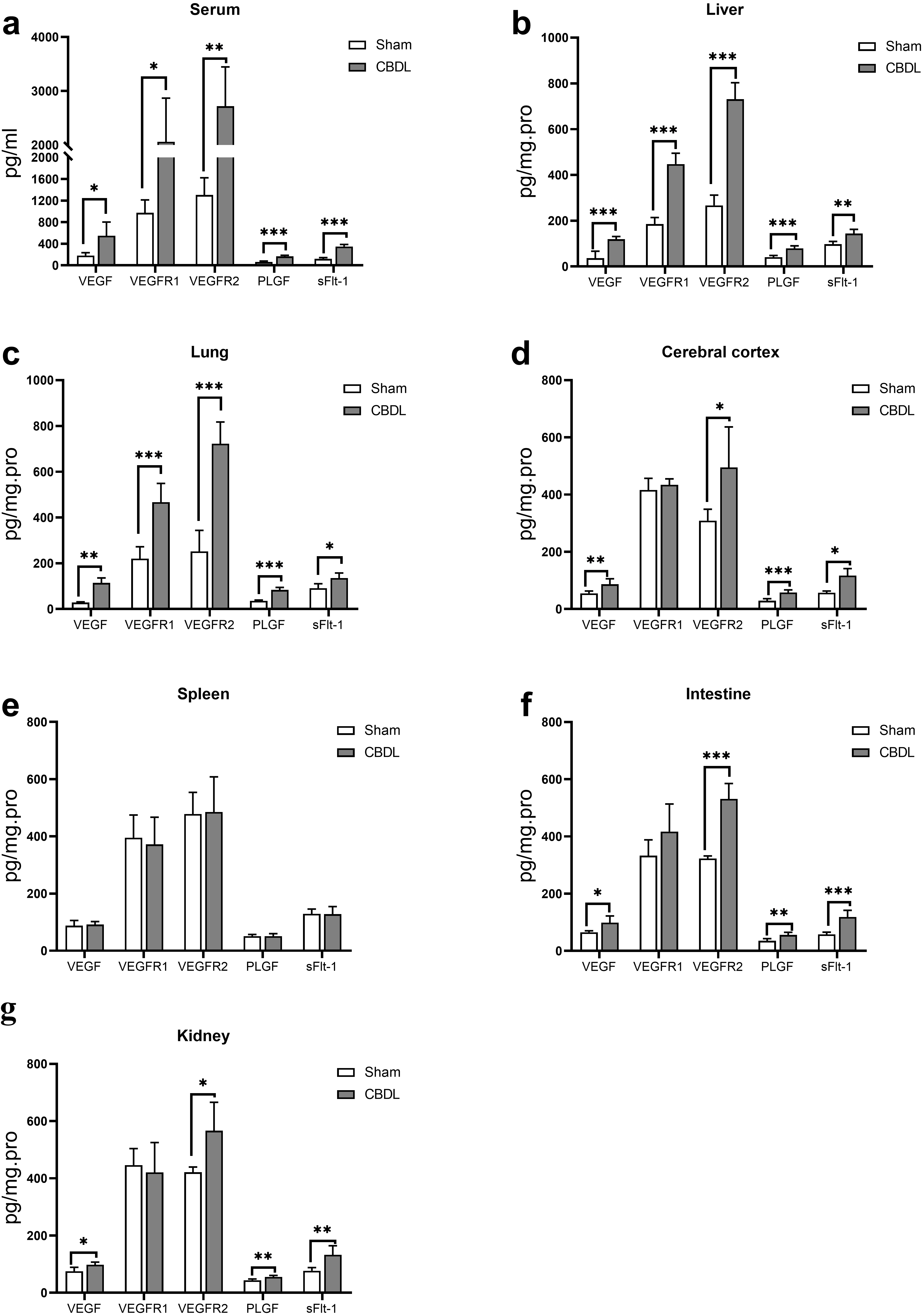
Expression level of VEGF, VEGFR1, VEGFR2, PLGF and sFlt-1 in
serum (a), liver (b), lung (c), cerebral cortex (d), spleen (e), intestine (f)
and kidney (g) of CBDL rats. Data were represented as mean
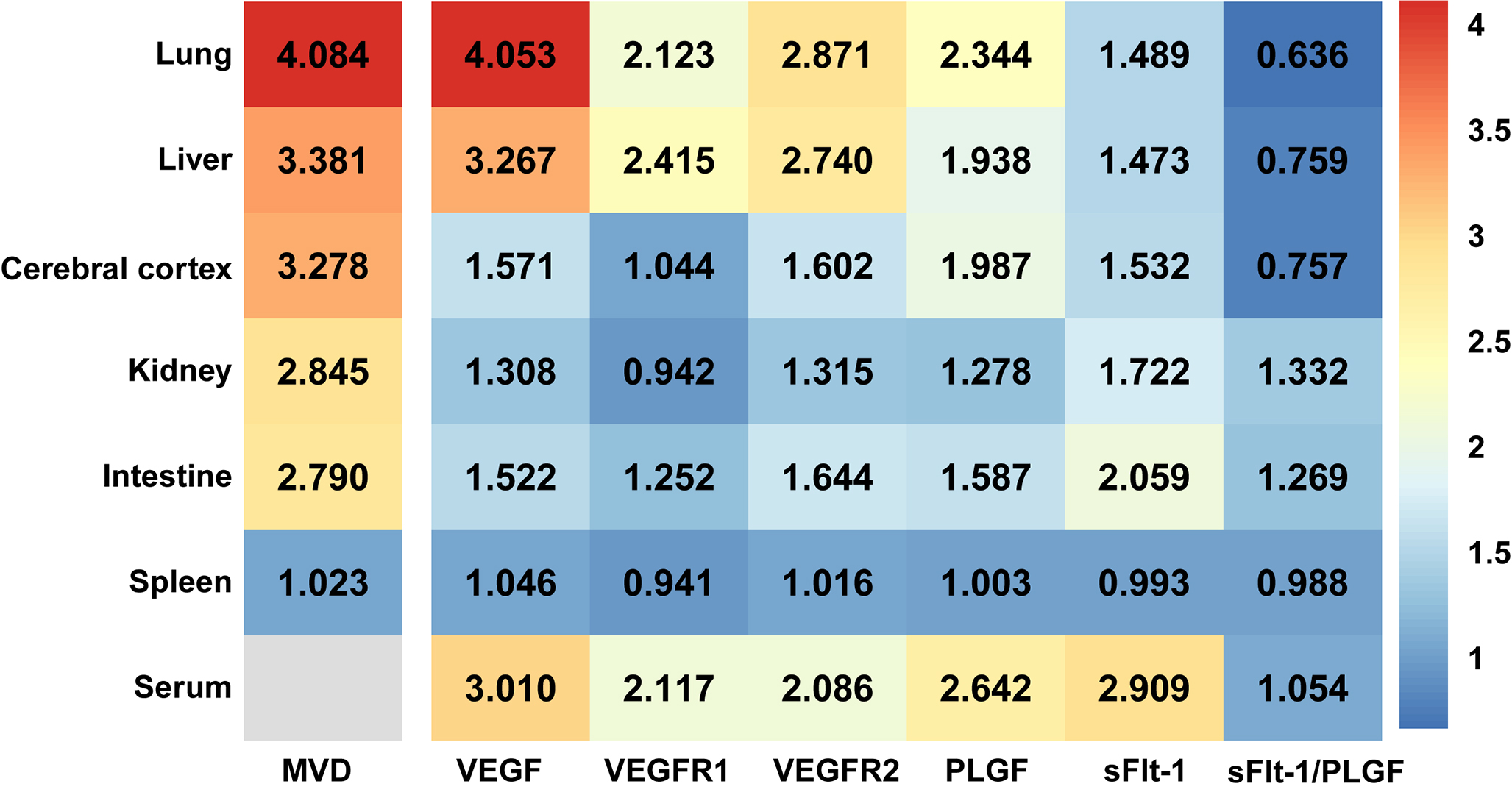
The MVD and fold change of expression level of VEGF family and its receptors in CBDL rats (sham group as baseline) presented by heatmap. MVD, Microvessel Density; VEGF, Vascular Endothelial Growth Factor; VEGFR1, Vascular Endothelial Growth Factor Receptor 1; VEGFR2, Vascular Endothelial Growth Factor Receptor 2; PLGF, Placental Growth Factor; sFlt-1, Soluble Vascular Endothelial Growth Factor 1; sFlt-1/PLGF, Soluble Vascular Endothelial Growth Factor 1/Placental Growth Factor.
PLGF was obvious up-regulated in rodent model of liver cirrhosis and cirrhotic patients, and PLGF played an important role in pathological angiogenesis in liver cirrhosis [30]. Recently, PLGF has also attracted intense interest as a possible antiangiogenic therapeutic target for treating HPS [17]. In our study, we noticed moderate levels of sFlt-1 in liver, lung, and cerebral cortex, whereas levels of PLGF increased significantly in the same tissues. Moreover, the ratio of sFlt-1/PLGF in kidney and intestine of CBDL rats was increased, while the ratio of sFlt-1/PLGF in the liver, lung, and cerebral cortex was decreased (Fig. 5). Interestingly, a reduction in the level of sFlt-1/PLGF ratio was accompanied by a more significant increase in MVD in the tissues. Our findings suggest that the sFlt-1/PLGF ratio might be a potential further study target for extrahepatic pathological angiogenesis in cholestatic cirrhosis.
Our current study has two main novel findings. First, extrahepatic angiogenesis may be a potential common pathophysiological basis for multiple organ dysfunction in rats with cholestatic cirrhosis (Fig. 6). Since the VEGF family varies in each organ, the degree of extrahepatic angiogenesis also varies. Second, the imbalance of pro-angiogenetic and anti-angiogenetic factors reflected by the sFlt-1/PLGF ratio may be a potential target for extrahepatic pathological angiogenesis in cholestatic cirrhosis.
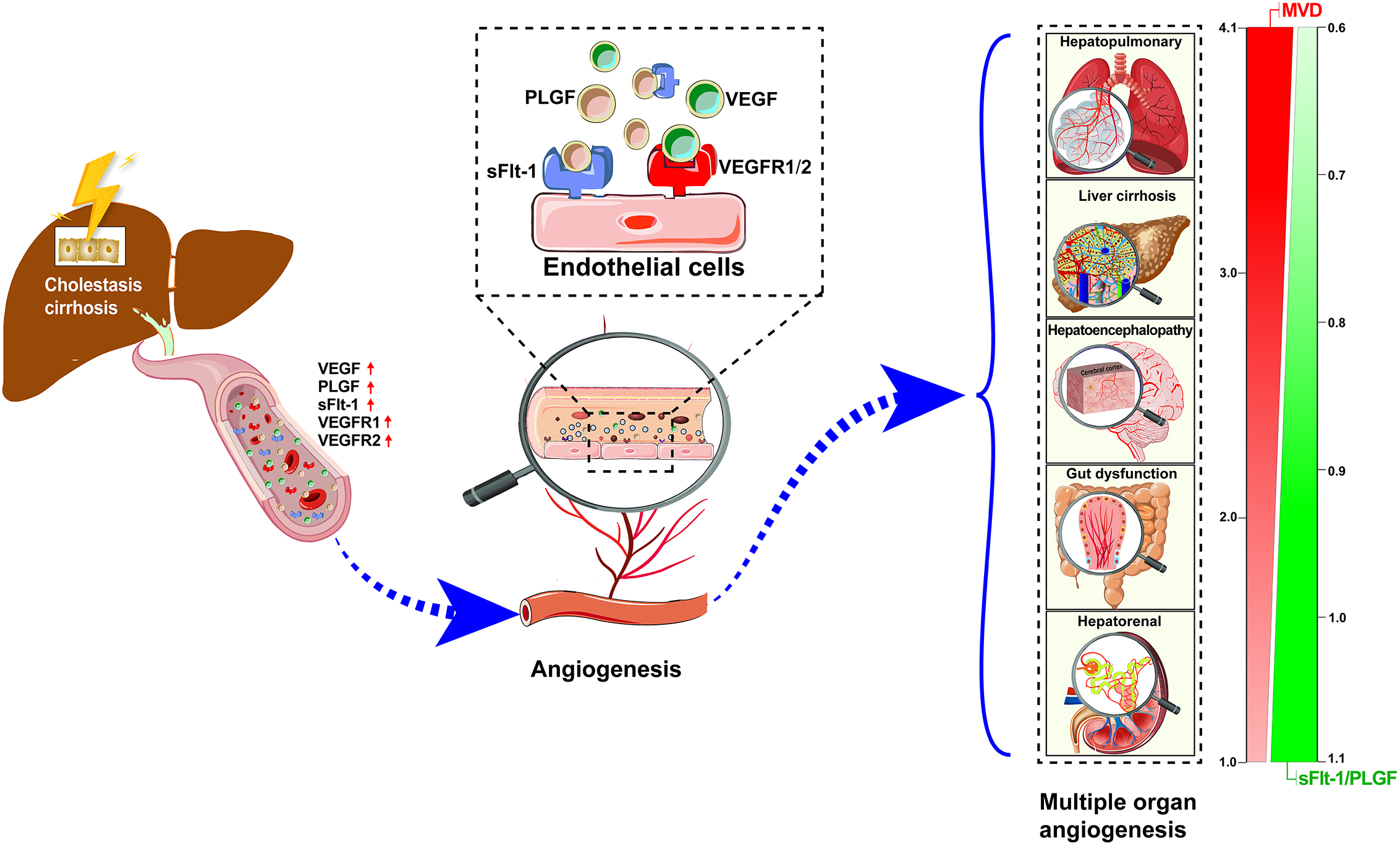
Hypothesis representation of pathological angiogenesis and VEGF family/receptor changes are one of the common pathophysiological mechanisms of extrahepatic multiple organ dysfunction in cholestatic cirrhosis. MVD, Microvessel Density; VEGF, Vascular Endothelial Growth Factor; VEGFR1, Vascular Endothelial Growth Factor Receptor 1; VEGFR2, Vascular Endothelial Growth Factor Receptor 2; PLGF, Placental Growth Factor; sFlt-1, Soluble Vascular Endothelial Growth Factor 1; sFlt-1/PLGF, Soluble Vascular Endothelial Growth Factor 1/Placental Growth Factor.
It is well recognized that hemodynamic disorders associated with PH in liver cirrhosis contribute to the development of dysfunction of systemic organs [11, 13, 31]. The mechanisms of PH and associated extra-hepatic injuries are complex, in which pathological angiogenesis is accepted as one of the important mechanisms of hemodynamic disturbances in liver cirrhosis [13] and targeting only “pathological angiogenic activity” is promising for a novel approach to ameliorate cirrhotic-associated complications [5]. Our study showed that CBDL rats suffers multiple organ damage, with liver, lung and cerebral cortex injuries being the most pronounced, which is in line with similar reports [32]. These findings are consistent with previous clinical reports that patients with advanced liver cirrhosis often have pulmonary, cerebral and renal failure [33]. Moreover, we found that the more obvious the pathological angiogenesis in specific organ, the more severe injury occurred, especially in the liver, lung and cerebral cortex. These pathological changes may help to explain, to some extent, that extrahepatic angiogenesis is involved in multiple organ injury. In addition, the MVD of extrahepatic organs in CBDL rats exhibited significant organ-specific elevations, with the highest MVD in the liver, lung, and cerebral cortex, followed by the kidney and intestine, with no significant change in the spleen. The current evidences indicated that multiorgan angiogenesis as a potential mechanism besides hemodynamic disorders is involved cirrhotic multiorgan damage and complex mechanisms target on different organs with different emphasis. It is accepted that pulmonary angiogenesis is an important pathological process in the development of HPS [34]. In hepatic encephalopathy, chronic hypoxia cerebral stimulation secondary to redistribution of the cerebral blood flow may induce brain angiogenesis and vascular remodeling [35]. And in turn, angiogenesis has abnormally high blood-brain barrier permeability, might have a share in the pathogenesis of aggravating hepatic encephalopathy [36, 37]. Although hepatorenal syndrome (HRS) is not rare in cirrhotic patients, MVD is not altered as markedly as in the lung and the cerebral cortex in the CBDL rats. It may be related to the fact that HRS is induced by a cascade of events such as hemodynamic changes and collateral circulation [16], rather than just pathological angiogenesis. Overall, this phenomenon indicates that extrahepatic injury and pathological angiogenesis are common and tissue-specific, most notably in the lung and the cerebral cortex.
In the present study, we found that pathological angiogenesis has shared characteristics in regarded to injury to different organs: elevated VEGF family cytokines induce increased angiogenesis, leading to create rampant growth of blood vessels and organ dysfunction eventually (Fig. 6). In mammals, the VEGF family is composed of five members, VEGF-A to D and PLGF, which bind to their receptors promoting angiogenesis. VEGF-A (usually referred to as VEGF) is a trophic factor for both physiological and pathological angiogenesis, whereas PLGF is used only for pathological angiogenesis. The most important receptors for VEGF and PLGF are VEGFR1, VEGFR2, and sFlt-1. An up-regulation of VEGF family was previously reported in animal models as well clinical studies and linked with ongoing angiogenesis in HPS and other extrahepatic tissues in the setting of liver cirrhosis [30]. Indeed, Raevens et al. [17] found that elevated levels of PLGF in the lung was also responsible for the development of HPS, and neutralization of PLGF with antibodies ameliorated HPS by counteracting pulmonary angiogenesis. Genetic knockout of PLGF or inhibition of PLGF activity resulted in reduction of angiogenesis and liver fibrosis [38]. In our model, CBDL rats were accompanied by increased pathological angiogenesis and significantly increased levels of VEGF, VEGFR2, and PLGF in multiple extrahepatic organ, with the consistent with previous studies [11, 26]. sFlt-1 was also significantly elevated in the liver, lung, cerebral cortex, kidney, and intestine with increased MVD. As an endogenous anti-angiogenetic factor, sFlt-1 binds to VEGF and PLGF to negatively regulate their activity, and elevated sFlt-1 levels are tied to worse prognosis in sepsis, preeclampsia, and cirrhosis [39].
Our study also provides novel data on the relationship between sFlt-1 and PLGF serum levels as well as well as the correlation between the ratio of sFlt-1/PLGF and pathological angiogenesis. It has been found that the serum levels of sFlt-1 and PLGF are inversely correlated with hepatic and pulmonary function [40, 41], further supporting sFlt-1 and sFlt-1/PLGF as promising markers for diseases. In regards to pathological angiogenesis, experimental data suggest that sFlt-1/PLGF was significantly decreased in HPS rat liver, lung, and cerebral cortex, which are the tissues with the most increased MVD. Conversely, although kidney and intestine injuries were accompanied by increased MVD, sFlt-1/PLGF was significantly elevated. It thus may suggest that the pathological angiogenesis mechanism in the liver, lung and cerebral cortex is different from that in the kidney and intestine, and indicate that cirrhosis-associated kidney and intestine injures have other important mechanisms (which causes other well-known sequelae of portal hypertension and hyperdynamic circulation) besides pathological angiogenesis. Our results demonstrated that changes in the VEGF family and its receptors also vary in different extrahepatic tissues, following tissue-specific injury and angiogenesis. Moreover, sFlt-1/PLGF may be one of the key factors for pathological angiogenesis in the liver, lung, and cerebral cortex, and is expected to be a promising target for extrahepatic angiogenesis in liver cirrhosis. Importantly, as liver cirrhosis is characterized by multiorgan angiogenesis with elevated circulating sFlt-1and PLGF, total PLGF can now be easily calculated from already available free PLGF and sFlt-1 levels, allowing subsequent evaluation of PLGF alternation in cirrhotic situation [42].
Our study has some limitations. First, we only reported an interesting phenomenon, that is, angiogenesis in extrahepatic organs (the lung, kidney, brain, and intestine, etc.) accompanied by injury in CBDL rats; however, no intervention studies were performed here to explain their causal relationship. Despite the well-defined pathophysiological roles of sFlt-1 and PLGF, the underlying molecular and cellular mechanisms are not completely understood, especially the exact relationships between biochemical events and molecular pathways regulated by sFlt-1 and PLGF, whose inhibition exhibits a protective role in cirrhotic multiorgan syndrome. Second, in our study, this is only an animal experiment, and clinical observations are required for practice guidance.
Taken together, the pathophysiological hallmark of liver cirrhosis is the presence of angiogenesis, which can involve multiple affected organs, such as the lung, kidney, brain and intestine. Extrahepatic angiogenesis may be a potential common pathophysiological basis for multiple organ dysfunction induced by cholestasis cirrhosis. Meanwhile, sFlt-1/PLGF ratio may be a potential further study target for extrahepatic pathological angiogenesis in cholestatic cirrhosis.
The datasets used and/or analyzed during the current study are available from the corresponding author on reasonable request.
BY and XW conceived of and supervised the study. KB supervised the study and provided necessary guidance in data interpretation and writting. DW, CY and YL conducted the experiments and wrote the manuscript. ZZ, XW, JL, HL and XH collected the data. YS, JL, XS and ZY analysed and interpreted the data. All authors contributed to editorial changes in the manuscript. All authors read and approved the final manuscript. All authors have participated sufficiently in the work to take public responsibility for appropriate portions of the content and agreed to be accountable for all aspects of the work in ensuring that questions related to its accuracy or integrity.
The ethical committee for animal care (AMUWEC20201507) approved all animal protocols. There is no informed consent because there is no patient involved in this study.
We thank all the laboratory technicians who participated in this study.
The work was supported by National Science Foundation of China (Grant No. 82100658 and 82070630), Special support for Chongqing postdoctoral research project in 2020, Chongqing Science and health joint medical research project (Grant No. 2020FYYX076), and special support project for improving scientific and technological innovation ability of undergraduate (Grant No. 2021XBK19).
The authors declare no conflict of interest.
Publisher’s Note: IMR Press stays neutral with regard to jurisdictional claims in published maps and institutional affiliations.