- Academic Editor
Ischemic stroke is one of the major causes of death and disability. Since the currently used treatment option of reperfusion therapy has several limitations, ongoing research is focusing on the neuroprotective effects of microglia and stem cells. By exerting the bystander effect, secreting exosomes and forming biobridges, mesenchymal stem cells (MSCs), neural stem cells (NSCs), induced pluripotent stem cells (iPSCs), and multilineage-differentiating stress-enduring cells (Muse cells) have been shown to stimulate neurogenesis, angiogenesis, cell migration, and reduce neuroinflammation. Exosome-based therapy is now being extensively researched due to its many advantageous properties over cell therapy, such as lower immunogenicity, no risk of blood vessel occlusion, and ease of storage and modification. However, although preclinical studies have shown promising therapeutic outcomes, clinical trials have been associated with several translational challenges. This review explores the therapeutic effects of preconditioned microglia as well as various factors secreted in stem cell-derived extracellular vesicles with their mechanisms of action explained. Furthermore, an overview of preclinical and clinical studies is presented, explaining the main challenges of microglia and stem cell therapies, and providing potential solutions. In particular, a highlight is the use of novel stem cell therapy of Muse cells, which bypasses many of the conventional stem cell limitations. The paper concludes with suggestions for directions in future neuroprotective research.
There are over 12 million stroke cases each year worldwide and over 6.5 million people die from stroke annually [1]. According to DALY (disability-adjusted life year), which is an indicator that measures the overall burden of the disease [2], more than 143 million years of healthy life are lost annually due to stroke-related death and disability [1]. As reported in the thirty-year projections of stroke incidence, prevalence, deaths, and disability-adjusted life years, there was a 27% increase in the number of people living with a stroke estimated between 2017 and 2047 in the European Union [3].
Current methods of reperfusion therapy include intravenous thrombolysis and mechanical thrombectomy. However, they are associated with several adverse effects, such as the risk of intracranial hemorrhage, allergic reactions, hypotension, risk of bleeding, acute kidney injury [4], emboli, vessel dissections, and vasospasms [5], while also having narrow therapeutic time windows [6], which means that only a small percentage of stroke patients are able to benefit from such treatment [7]. Therefore, there is a great need for the development of neuroregenerative and neuroprotective methods.
The occlusion of cerebral and precerebral arteries caused primarily by either
atherosclerotic plaques in large vessels, microatheromatosis, or cardioembolism
[8] leads to anoxia and activation of anaerobic metabolism, which causes the
formation of lactic acid and contributes to the dysregulation of the acid–base
balance and cell destruction [9]. Reduced adenosine triphosphate (ATP) production leads to the impaired
function of ion pumps, the outflow of K
Until the development of photon imaging, genome-wide transcription analysis, and
epigenetic analysis, microglia were classified into two main phenotypes [15]:
classically activated M1 with proinflammatory properties that release tumor
necrosis factor alpha (TNF-
Neurogenesis involves the proliferation of neural stem cells, migration of neuroblasts, differentiation of neuroblasts into neurons, development of synaptic connections with other neurons, and survival of immature and mature neurons [7, 23]. In an adult brain, neurogenesis takes place mainly in two regions: in the subgranular zone of the hippocampal dentate gyrus (SGZ) and in the subventricular zone (SVZ) along the lateral ventricles [24].
Neurogenesis can be stimulated by an ischemic episode. Unfortunately, most neurons formed in this way die within about 2 weeks of a stroke. The low survival rate of new neurons is thought to be due to a lack of trophic factors and chronic neuroinflammation [24].
One of the factors stimulating neurogenesis in an adult brain is vascular endothelial growth factor (VEGF), which inhibits apoptosis of hippocampal neurons, stimulates stem cell proliferation and migration of newly formed cells via the vascular endothelial growth factor receptor 2 (VEGFR2) pathway, and stimulates angiogenesis and the repair of damaged neurons [25]. The stroke-induced blood–brain barrier disruption facilitates the contact between adult neural stem cells (NSC) and vascular cells, including VEGF. It was shown that VEGF induces the expression of the Notch ligand Delta-like 4 (DLL4) via its receptor VEGFR2, which leads to the proliferation and differentiation of NSCs into neurons [26]. Angiopoietin-like 4 (ANGPTL4) protein, which is released by vascular cells as a result of hypoxia, has been shown to stimulate neurogenesis in the dentate gyrus of the hippocampus and subventricular zone by stimulating Akt kinase activity and it also reduces the inflammatory response and neuronal death by inhibiting Fas expression and the Fas ligand (FasL) [27]. Fibroblast growth factor 2 (FGF-2) stimulates the proliferation and differentiation of neural progenitor cells derived from the subventricular zone. It was shown that the infusion of FGF-2 into the rats’ lateral ventricles increased the proliferation and migration of neurons from the subventricular zone to the olfactory bulb, and the injection of FGF-2 neutralizing antibodies contributed to the inhibition of their proliferation. Moreover, fibroblast growth factor also stimulates post-ischemic neurogenesis in classically non-neurogenic areas, such as the striatum, substantia nigra, and cerebral cortex [28]. The stromal cell-derived factor 1 and C-X-C chemokine receptor type 4 and 7 (SDF-1/CXCR4/CXCR7) signaling pathway stimulates axonal elongation and branching, remyelination, and the migration, proliferation, and differentiation of neuronal progenitor cells [29]. Moreover, insulin-like growth factor 1 (IGF-1) and brain-derived neurotrophic factor (BDNF) also enhance stem cell proliferation. Monocyte chemotactic protein (MCP-1) and matrix metalloproteinases 2, 3, and 9 (MMP) stimulate the migration of neuroblasts [30].
Although initially it was proposed that transplanted stem cells directly replace
neurons in ischemic regions, currently, it is postulated that their therapeutic
effect is mainly the result of their paracrine action (the “bystander effect”)
since most of the systemically injected stem cells are trapped in the lungs and
do not reach the affected tissues [31]. The paracrine action involves the
secretion of factors that stimulate endogenous neurogenesis (BDNF, FGF,
angiopoietin 2), angiogenesis (VEGF, angiopoietin 2), and neuroplasticity
(integrin
In 2013, a new mechanism through which transplanted stem cells exert their therapeutic functions was proposed. Exogenous cells were found to form “biobridges” between the neurogenic area (subventricular zone, SVZ) and the ischemic area, thereby facilitating the successful migration of endogenous stem cells, which is one of the key limitations in the endogenous repair system. Biobridges, consisting of metalloproteinases (MMP) and an extracellular matrix (ECM), form a pathway that helps direct the migration of endogenous stem cells to the damage zone through non-neurogenic brain areas [40]. Increased activity of MMP-9 along the formed biobridges was demonstrated, and its inhibition was shown to impair cell migration from the SVZ to the cerebral cortex, suggesting a key role of metalloproteinase 9 in ECM remodeling. Interestingly, once the exogenous stem cells form biobridges, their concentration decreases and they are replaced by endogenous cells derived from neurogenic areas of the brain; thus, making their long-term administration potentially unnecessary [41]. Moreover, an increase in endogenous cell proliferation and neural differentiation in the peri-injured cortical areas was demonstrated, which further suggests that the transplantation of exogenous stem cells and biobridge formation can facilitate endogenous repair mechanisms. As mentioned before, ischemia-induced endogenous post-stroke neurogenesis itself is insufficient because of low stem cell survival and migration rates, incomplete integration in neural circuits, and increased differentiation to glial cells [42]. However, more studies explaining the mechanisms underlying the migration pathways and their implications in stroke therapy are still needed.
The stem cells most frequently used in medical research are mesenchymal stem
cells [43], which exhibit several properties that make them suitable for cell
transplants in stroke therapies [44]. They are multipotent, meaning they can
differentiate into more than one cell type, including mesodermal lineage
adipocytes, chondrocytes, osteocytes, and ectodermal lineage cells, such as
neurons and glial cells [45]. They are relatively easy to harvest because they
can be obtained from various body tissues, such as adipose tissue, bone marrow,
umbilical cord blood, umbilical cord tissue, dental tissue, and olfactory mucosa,
while their isolation and amplification are not expensive [43]. They can be
injected in several ways: intracerebrally, cerebroventricularly, intravenously,
intra-arterially, or intranasally [43]. MSCs exhibit immunomodulatory properties
by reducing the expression of proinflammatory cytokines, such as TNF-
The current microglia research focuses on preventing the excessive
activation and production of proinflammatory molecules [51]. One of the
therapeutic strategies for ischemic stroke uses oxygen–glucose deprivation
(OGD), whereby an optimal ischemia event is hypothesized to induce the protective
phenotype in microglia [52]. Intravascular administration of OGD-preconditioned
microglia in animal models was shown to promote angiogenesis, axonal outgrowth,
and functional recovery. Since the main outcome of the microglial activity is
considered to coincide with the result of the secreted neurotrophic factors
rather than the microglial cells themselves, the effect on the neurological
recovery by extracellular vesicles (EVs) derived from OGD preconditioned
microglia was investigated. EVs from OGD-preconditioned microglia were found to
be high in TGF-
Other factors affecting microglia activation phenotypes include IL-4 and
IFN-
A variety of other factors that promote anti-inflammatory properties by
microglia are being researched. Minocycline, an antibiotic from the tetracycline
group was shown to increase the survival of neurons, stimulate neurogenesis,
inhibit reactive gliosis, and promote functional recovery via the STAT1/STAT6
pathway in a rodent study model [59]. IL-4 was associated with improved
functional recovery after stroke and a deficit in endogenous IL-4 promoted the
proinflammatory phenotypic conversion [60]. Recently, tetramethylpyrazine, used
in treating cerebrovascular disorders, was shown to modulate microglial
polarization via the JAK2–STAT1/3 and GSK3–NF
Stem cell-based therapies for ischemic stroke using mesenchymal stem cells (MSCs), neural stem cells (NSCs), induced pluripotent stem cells (iPSCs), and multilineage-differentiating stress-enduring cells (Muse cells) have been extensively researched recently. Indeed, stem cells can exert immunomodulatory, proangiogenic, and proneurogenic functions through their paracrine action, exosome secretion, and biobridge formations.
Because the main mechanism through which mesenchymal stem cells exhibit their
paracrine properties is exosome secretion [34] and extracellular vesicles (EVs)
derived from MSCs have been associated with promising therapeutic results in
rodent stroke models [64, 65, 66, 67, 68, 69, 70, 71], current research has been focusing on elucidating
the mechanisms of their action. Exosomes contain various proteins, including
cytokines, chemokines, growth factors, and membrane receptors as well as miRNAs
through which they promote neurogenesis, angiogenesis, and cell growth and reduce
inflammation, oxidative stress, and cell death [72, 73, 74, 75]. Different miRNAs are
carried in MSC-derived exosomes and target different mechanisms involved in
stroke. It has been demonstrated that exosomes enriched with miRNA-17–92
increased the proliferation of neural and oligodendrocyte progenitor cells and
increased neural plasticity via the PI3K/Akt/mTOR/GSK-3
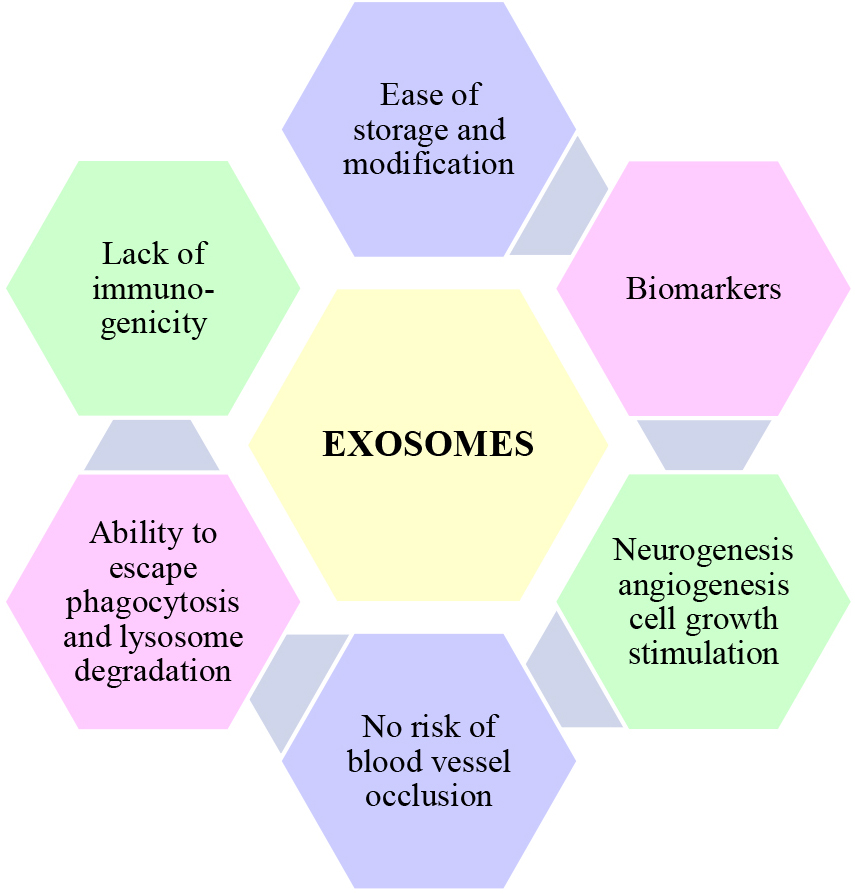
Exosome characteristics. Exosomes contain various proteins, including cytokines, chemokines, growth factors, and membrane receptors as well as microRNAs through which they promote neurogenesis, angiogenesis, and cell growth and reduce inflammation, oxidative stress, and cell death. They have the ability to pass through the blood–brain barrier and escape phagocytosis and lysosome degradation. Their advantages over cell therapies include ease of storage and modification and no risk of blood vessel occlusion. As their miRNA content varies in relation to the progression of stroke, they can be used as biomarkers.
While stem cell therapies were assessed to be relatively safe, with low risks of tumorigenesis [103] and the association of only minor adverse effects [104], the results in terms of their efficacy are mixed. Several studies did not show any significant difference between the treatment and control group [105, 106, 107]. Moreover, although many clinical trials demonstrated improvements in neurological functions [108, 109, 110, 111, 112], the studies yielding positive results presented high risks of selection, performance, and publication bias and had small sample sizes [113]. In addition, in most cases, they lacked an effective study design, including randomization, blinding, and statistical comparison [104]. Interestingly, the unsatisfactory results for efficacy can be explained by differences between the preclinical and clinical protocols [114]. In several trials, doses below the efficacious dose previously established in preclinical studies were used [106, 115]. Taking into consideration the above limitations of recent clinical trials, more studies with larger sample sizes, longer follow-ups, improved methodological designs, and better adherence to preclinical outcomes are needed.
In addition to mesenchymal stem cells, neural stem cells (NSCs) are also used in stroke research. However, their harvesting methods are problematic. Their sources are limited and transplantation from the adult brain would require complicated surgery. Derivation from neuroectoderm of the fetal tissue or from embryonic stem cells (ESCs) raises major ethical concerns and such transplantations may result in tumor formation [116, 117]. Generating NSCs from induced pluripotent stem cells (iPSCs) [118] or via direct neuronal reprogramming by omitting the PSC stage would eliminate any ethical issues and the risk of immune rejection because they can be obtained from the patient’s own cells [119, 120]. However, the reprogramming process is long and time-consuming and iPSC transplantations in animal models have been associated with tumor formation [121]; thus, improving their safety remains the primary issue [122]. Moreover, as their engraftment efficiency is low [123], their therapeutic effects are achieved mainly by the paracrine action, including secreting factors enhancing neurogenesis, angiogenesis, and reducing inflammatory responses [119]. Thus, the current research involving neural stem cells focuses primarily on the extracellular vesicles derived from NSCs that present lower tumorigenicity, improved blood–brain barrier (BBB) permeability, and biodistribution. The comparison of extracellular vesicles (EV) derived from neural stem cells and mesenchymal stem cells (both derived from the same pluripotent stem cell line) in animal stroke models demonstrated a higher effectiveness of NSC treatment. Therapy using NSC EVs resulted in a larger reduction in infarct size, greater improvement in somatosensory function, and smaller neurological deficits than after treatment with MSC EVs. Moreover, therapy using NSC EVs was associated with an increase in macrophages with protective phenotypes and a decrease in proinflammatory T helper 17 cells (Th17) [124]. Although the initial results are encouraging, more research studies elucidating the downregulation of inflammatory responses are still needed.
Interestingly, mesenchymal stem cells used in combination with neural stem cells have been proven more effective in animal models than the use of individual therapies [125], while the co-transplantation of MSCs and NCSs in stroke patients has been shown to be a safe and feasible method [112], making it a potential new therapy for ischemic stroke patients [126]. Furthermore, stem cell therapy has also been shown to be effective in combination with gene therapy [127, 128], tissue engineering scaffolds [129, 130, 131, 132], and reperfusion therapy [114].
Cell therapy using multilineage-differentiating stress-enduring cells (Muse cells) appears to overcome a large number of limitations by MSCs, NSCs, iPSCs, and ESCs (Table 1). Muse cells, first reported in 2010 [133], are found in a variety of tissues, such as bone marrow, peripheral blood, connective tissue, and the umbilical cord [134]. They can differentiate into three germ layers, including spontaneous in vivo differentiation into neuronal cells [135] and they can integrate into the neural network [136]. Moreover, they exhibit paracrine functions by secreting a variety of neurotrophic, proangiogenic, anti-inflammatory, and antiapoptotic factors. They are immune-privileged, meaning they inhibit the inflammatory immune response by, as suggested, expressing human leukocyte antigen G (HLA-G) molecules, meaning HLA-matching or immunosuppressant treatment is not required [134]. Due to the high expression of sphingosine-1-phosphate receptor 2 (S1PR2), as part of the S1P-S1PR2 axis, they can selectively migrate to the damaged site [137]. In addition, owing to their high capacity for DNA repair and lower telomerase activity and gene expression of tumorigenic factors than in ESCs and iPSCs, Muse cells are considered non-tumorigenic [134]. Muse cells have been researched in several animal stroke models [135, 138, 139, 140, 141] and were shown to differentiate into neuron cells, integrate into the cortex, improve motor functions and survival rates, and be assessed to be a safe treatment option. Moreover, in-human transplantations of allogenic Muse cells demonstrated safety and efficacy in clinical trials on myocardial infarction and dystrophic epidermolysis bullosa [142, 143]. However, there are several challenges associated with culturing Muse cells, whereby expanding their small populations is time-consuming and their culture cost is higher than that of other stem cell types. Moreover, golden standards with regard to cell sources, sorting methods, and donor age still have to be established [137] alongside more preclinical and clinical studies that investigate and illustrate their mechanisms of action.
Stem cells | Advantages | Disadvantages |
Mesenchymal (MSCs) | Easy cell harvesting | Decreased proliferation over time |
Inexpensive isolation and amplification | ||
Low risk of tumorigenesis | ||
Neural (NSCs) | Differentiates into all neural lineages | Limited sources |
Embryonic (ESCs) | Differentiates into three germ layers | Ethical issues |
Risk of tumorigenesis | ||
Induced Pluripotent (iPSCs) | Renewable source for stem cell therapy | Long reprogramming process; risk of tumorigenesis |
Multilineage-differentiating stress-enduring cells (Muse cells) | Differentiate into three germ layers | Few original papers published |
Non-tumorigenic | ||
High homing capacity | ||
Immune-privileged |
Stroke remains one of the leading causes of death and disability. The administration of stem cells and preconditioned microglia has been associated with various neuroprotective and immunomodulatory effects in preclinical studies. However, many challenges remain in cell therapy that need to be overcome.
The underlying mechanisms of their action are still not fully understood and various signaling pathways and phenotypic cell markers still need to be researched alongside their therapeutic implications established.
Moreover, cell therapy presents several important translational challenges. Preclinical studies performed in vitro cannot accurately mirror intricate brain environments and cellular interactions with various factors in an ischemic brain. In addition, cell sources should be also considered—for example—mouse microglia used in animal model studies present differences from human microglia. Importantly, golden standards in clinical trials regarding the dosage, administration route, time window after a stroke, cell source, and adverse event management systems should be established along with larger samples, control groups, longer follow-ups, and improved methodological designs to further study the safety and efficacy of cell therapy. Furthermore, more research on the combined use of stem cells with reperfusion methods, gene therapy, tissue scaffolds, and different types of stem cells should be conducted.
Although an overall cure for ischemic stroke has still not been found, recent advances in cell therapies and a growing understanding of their underlying mechanisms represent a promising start to achieving an effective neuroregenerative and neuroprotective treatment.
AM, Ideation, literature search and writing the manuscript; DK and SS, work revision and suggestions to “stroke pathophysiology” chapter. All authors contributed to editorial changes in the manuscript. All authors read and approved the final manuscript.
Not applicable.
Not applicable.
This research received no external funding.
The authors declare no conflict of interest.
Publisher’s Note: IMR Press stays neutral with regard to jurisdictional claims in published maps and institutional affiliations.