- Academic Editor
†These authors contributed equally.
The oral cavity serves as the initial segment of the digestive system and is responsible for both nutritional supplementation and the mechanical breakdown of food. It comprises distinct hard and soft tissues; the oral mucosa is subject to mechanical stress and interaction with microbiota. In oral cancer, tumors exhibit abnormal cellular networks and aberrant cell-cell interactions arising from complex interplays between environmental and genetic factors. This presents a challenge for clinicians and researchers, impeding the understanding of mechanisms driving oral cancer development and treatment strategies. Lesions with dysplastic features are categorized under oral potentially malignant disorders, including oral leukoplakia, erythroplakia, oral submucous fibrosis, and proliferative verrucous leukoplakia, carrying a high malignancy risk. In this review, we discuss oral cancer cell characteristics and the stiffness of the surrounding matrix. We also discuss the significance of stiffness equilibrium in oral potentially malignant disorders, particularly oral submucous fibrosis, possibly triggered by mechanical stress such as betel quid chewing.
Cancer cells manifest six distinct biological characteristics that are acquired throughout the multistage development of human tumors. These include (1) the sustenance of growth signaling, (2) the evasion of growth inhibitors, (3) cell death resistance, (4) replication immortality, (5) initiation of angiogenesis, and (6) the activation of infiltration and metastasis. Recent conceptual progress has supplemented this list with two additional traits: energy metabolism reprogramming and evasion of immune destruction. These features serve as foundational principles that streamline the complex nature of neoplastic diseases. These features are characterized by genomic instability, generating genetic diversity, which consequently facilitates their acquisition, as well as inflammation that contributes to diverse characteristics and functions [1]. In this context, various stresses, such as oxidative stress [2] and matrix stiffness [3], inevitably connect them, thereby complicating the determination of clinical cancer progression and outcome. Mechanical signaling triggers mitochondrial and metabolic reprogramming, enabling cells to adapt to oxidative stress [4]. Matrix stiffness is a critical factor in cancer progression and is caused by the accumulation, contraction, and cross-linking of the extracellular matrix (ECM) by cancer and stromal cells. These cells respond to matrix stiffness, which determines their phenotype. Furthermore, matrix stiffness activates and/or deactivates specific transcription factors within cancer and stromal cells, consequently regulating cancer progression [5].
Oral submucous fibrosis (OSMF) is an oral potentially malignant disorders
(OPMDs) [6]. Betel quid (BQ) chewing serves as a potential risk factor,
exacerbating the propensity for oral cancer and OSMF, a premalignant oral
condition with malignant transformation potential. The constituents of BQ, such
as areca nuts, coarse areca nut fiber-induced trauma, catechin, copper,
alkaloids, reactive oxygen species (ROS) induction, inflammation, and
cytotoxicity, are likely contributory factors [7, 8]. These components
potentially incite tissue inflammation, stimulate fibroblast proliferation,
induce collagen deposition, trigger myofibroblast differentiation and
contraction, promote collagen cross-linking, and hinder collagen phagocytosis,
ultimately culminating in OSMF and oral cancer. These processes are orchestrated
by changes induced by BQ components in collagen-related factors, such as lysyl
oxidase (LO) [9]. Moreover, BQ components exhibit genotoxicity, potentially
altering DNA, protein, and lipid structures, resulting in antigen production.
Additionally, BQ components induce keratinocyte inflammation by stimulating the
production of prostaglandins, TNF-
Tumor cells encounter diverse mechanical stimuli within the tumor microenvironment, including cell-cell and cell-ECM tension, compression stress from expanding tumor masses, interstitial fluid pressure, and shear stress. These stimuli exhibit a positive feedback relationship with cellular responses, such as heightened actomyosin contractility and stiffening of the extracellular matrix, which exacerbate tumor progression and aggressiveness. A comprehensive understanding of the interplay between the components of the tumor microenvironment and the molecular mechanisms governing cellular reactions to mechanical inputs is pivotal for developing efficacious cancer treatments [12].
Metastasis is a pivotal aspect of cancer progression. Tumor cells metastasize from the primary tumor to distant organs, resulting in a life-threatening condition. Distinct biomechanical properties are responsible for tumor cell growth, invasion, intravascular invasion, circulation, arrest/adhesion, and extravascular invasion, collectively constituting the metastatic cascade. The basement membrane (BM) is a specialized extracellular matrix that serves as a major barrier that cancer cells must repeatedly overcome to metastasize [13].
Fiore et al. [14] proposed that mechanical forces exerted both above and below the progenitor cells in multilayer epithelia augment the formation of precancerous tumor structures that significantly impact tumor progression. Cellular transformation accompanies molecular changes altering cytoskeletal organization; therefore, cell stiffness must be evaluated to potentially facilitate the detection and assessment of cancer cells [15]. The ECM is the critical component of tumors, serving diverse roles, including mechanical support, microenvironmental modulation, and generation of signaling molecules. The quantity and cross-linking status of ECM components primarily determine tissue stiffness. During tumorigenesis, interactions between cancer cells and the tumor microenvironment (TME) frequently result in ECM rigidity, inducing aberrant mechanotransduction and further malignant transformations. A comprehensive understanding of ECM dysregulation within the TME is instrumental in identifying promising targets for cancer therapy [16]. Thus, stiffness exerts its influence on cellular components and the ECM in various regional cancers, including oral cancers [17, 18].
Metastatic cells typically exhibit lower stiffness than non-malignant tumor cells, with the high deformability of both the cell and its nucleus presumed to confer a significant advantage for their metastatic potential. However, the question of whether there exists a finely calibrated yet fixed mechanical state accommodating all requisite mechanical attributes for metastatic cell survival across the cascade or whether tumor cells dynamically refine their properties and intracellular components upon encountering each new stage remains unresolved [19]. Engler et al. [20] have demonstrated the impact of matrix elasticity on human mesenchymal stem cell (MSC) differentiation, yielding valuable insights into the physical effects of the in vivo microenvironment and the therapeutic potential of MSCs. Stiffness regulation extends beyond MSCs to encompass various cell types. In the vascular microenvironment, stiffness and fluid shear stress engage in a signaling interplay, with matrices mirroring the properties of young, healthy vessels effectively inducing fluid shear stress-mediated atheroprotection. These findings imply that targeting intimal stiffening and/or the endothelial cell response to intimal stiffening might enhance vascular health [21]. As tumor progression advances, the composition and physical attributes of the ECM undergo alteration. Elevated matrix stiffness notably influences tumor growth and metastasis. In particular, endothelial cells play a pivotal role in cancer progression; however, the impact of tumor stiffness on the endothelium and its metastasis-related effects remain unexplored. While stiffness is a trigger for malignant transformation, other contributing factors should not be overlooked.
OPMDs frequently precede oral cancer. Wang et al. [22] examined the rate and duration of malignant transformation among different OPMDs in a patient cohort. Epithelial dysplastic lesions exhibited a 1.89-fold higher risk of malignant transformation compared with that of non-dysplastic lesions. The anatomical site of OPMDs and the presence of epithelial dysplasia demonstrated significant associations with malignant transformation. Tongue lesions carried a hazard rate ratio 1.87 times higher than that for buccal lesions. These findings highlight the need for prolonged clinical follow-up of patients with OPMDs, with histopathological evaluation serving as a vital predictor of cancer development, enabling the monitoring of potential malignant transformation [22].
The alteration and functional defects in lymphocyte populations have been
observed in different stages of oral cancer. Yeh et al. [23] focused on
the lymphocyte population and investigated whether CD4
The mechanism of OSMF and its potential transformation into OSCC have been explored in previous studies. Key molecules and abnormal RNAs that have substantial implications for disease onset, progression, detection, diagnosis, monitoring, and prognosis have been identified [24]. Consequently, advanced diagnostic techniques have been employed to predict disease progression and assess the risk of malignant transformation, thereby providing insights into the importance of early detection and prevention of OPMDs [25].
Using quantitative mass spectroscopy, Reid et al. [26] observed that
the matricellular protein CCN1/CYR61 is tightly regulated by endothelial cell
stiffness. This regulation prompts stiffness-induced CCN1 to trigger
FOXA2 bound to the CDH1 promoter augments the expression of E-cadherin, leading to diminished cancer cell migration. Elevated FOXA2 expression in oral cancer tissues was associated with heightened E-cadherin expression, decreased lymph node metastasis, and improved patient survival. This FOXA2–E-cadherin is involved in regulating oral cancer cell metastasis, thereby shedding light on the tumor-suppressive activity of FOXA2 in oral cancer [27]. CDH1 mutations have been identified by Er et al. [31] using next-generation sequencing in 50 formalin-fixed paraffin-embedded tumor specimens obtained from patients with oral squamous cell carcinoma. The causative factors triggering these phenomena within cadherin expression patterns and mutations warrant further investigation to confirm the role of mechanical stress regulation.
Numerous physical factors exert influence on tumor progression, including increased matrix deposition that augments tumor stiffness, matrix remodeling owing to forces from cancer cells and stromal fibroblasts, matrix cross-linking, increased cellularity, and the accumulation of both solid and stromal pressure. Mechanosensitive ion channels of the Piezo-type (PIEZO channels, including PIEZO1 and PIEZO2) have been identified as ion channels activated by mechanical stimuli [32]. The Hippo pathway, responsive to extracellular conditions, governs cell proliferation via the nuclear translocation of downstream effectors, yes-associated protein (YAP), and transcriptional coactivator with PDZ-binding motifs (TAZ), thereby inducing target gene transcription [33, 34]. Hippo/YAP signaling is potentially a therapeutic target for treating squamous cell carcinoma [35]. Xu et al. [36] have reported that the fibrotic matrix prompts mesenchymal transformation of epithelial cells in OSMF. Elevated stiffness of the fibrotic matrix in OSMF leads to increased proliferation and epithelial-mesenchymal transition (EMT) of mucosal epithelial cells, wherein the PIEZO1 and YAP axes play vital roles in mechanical sensing and signal transduction [36]. Hiemer et al. [37] demonstrated that nuclear YAP and TAZ, which are well-known mechanotransducers, stimulate OSCC cell proliferation, survival, and migration. Global gene expression profiles following YAP and TAZ knockdown demonstrated changes in the regulation of genes implicated in protumorigenic signaling, including those essential for cell cycle progression and survival. Notably, the transcriptional signature governed by YAP and TAZ strongly correlated with gene expression alterations observed in human OSCC identified by The Cancer Genome Atlas (TCGA), highlighting the central roles of YAP and TAZ in OSCC biology [37]. Hasegawa et al. [38] examined the impact of YAP signaling on OSCC tumorigenesis. Loss-of-function experiments utilizing siRNA and inhibitors, coupled with immunohistochemical analysis of tissue specimens from patients with OSCC, revealed the involvement of YAP signaling in OSCC cell proliferation. Furthermore, they discovered that the calcium channel PIEZO1 is a transcriptional target of YAP signaling, implying that elevated PIEZO1 expression facilitates PIEZO1 agonist-dependent calcium influx and OSCC cell proliferation. Immunohistochemistry revealed YAP overexpression in the nucleus and/or cytoplasm of tumor cells. While PIEZO1 and Ki-67 were frequently expressed in OSCC specimens, their presence was negligible in non-tumor areas. These findings suggest that the YAP/PIEZO1 axis fosters OSCC cell proliferation [38]. This pathway has been linked to tumorigenesis in OSCC and hepatocellular carcinoma while also correlating with poorer prognoses in breast, ovarian, and hepatocellular carcinoma [39, 40, 41].
Transient receptor potential vanilloid 4 (TRPV4), a physicochemical
stress-sensitive ion channel, plays a pivotal role in the nuclear translocation
of YAP/TAZ in response to matrix stiffness and TGF
Matrix stiffness plays a crucial role in oral tissues and other ECM stiffness, a
crucial mechanical attribute of the nucleus pulposus (NP) tissue, contributing to
intervertebral disc degeneration (IDD) pathogenesis. Wang et al. [45]
reported elevated PIEZO1 expression and increased ECM elasticity modulus in
degenerative NP tissues. Stiff ECM activates the PIEZO1 channel, leading to
elevated intracellular Ca
The physical characteristics of a tumor are intricately linked to its phenotype and pose challenges during treatment. Increased stiffness applies forces on cancer cells, intensifying tumor invasiveness and compromising therapeutic efficacy [46]. Although solid tumor metastasis arises from various genetic programs, the result is consistent. Tumor cells evade the primary tumor and disseminate to distant organs by overcoming a series of physical barriers [47]. Consequently, heightened physical pressure can augment stem cell tumor properties. Genetic regulation offers an effective approach to controlling stiffness. Genetic alterations in basement membrane stiffness increase membrane tension computationally and accelerate invasive squamous cell carcinoma progression in vivo. Mechanical forces exerted above and below the progenitors of multilayered epithelia may reportedly shape premalignant tumor architecture and influence tumor progression [14]. As cancer develops in the epithelium and often infiltrates the mesenchymal connective tissues, cancer-associated fibroblasts within the cancerous mesenchyme exhibit characteristics reminiscent of mesenchymal stem cells and play a pivotal role in the malignant transformation of oral mucosa through the regulation of T-cell proliferation [48].
Only a minority of patients with cancer robustly respond to immune checkpoint inhibitors. This limited success may partly be attributed to the dense ECM that forms a barrier for T-cells. By comparing five preclinical mouse tumor models with heterogeneous tumor microenvironments, Nicolas-Boluda et al. [49] investigated the rate of tumor stiffening, ECM structure remodeling, and the impact of these factors on intratumoral T-cell migration. A strategy targeting ECM through LO inhibition indicated that in vivo stiffness correlated positively with tumor growth and ECM cross-linking but negatively with T-cell migration. Furthermore, inhibiting collagen stabilization reduced ECM volume and tumor stiffness while boosting T-cell migration and enhancing the efficacy of anti-PD-1 blockade. These findings provide mechanistic insights into the characteristics of solid tumors and aid in understanding immunotherapy resistance and a rationale for integrating ECM-targeted therapeutic approaches with anti-PD-1 therapy [49].
LO, a copper-dependent amine oxidase, substantially contributes to the biogenesis of connective tissue matrices by cross-linking ECM proteins such as collagen and elastin. LO levels increase in numerous fibrotic conditions [50]. LO catalyzes the oxidative deamination of lysine residues in elastin and collagens, initiating their assembly into insoluble fibers within the extracellular matrix [51]. Trivedy et al. [52] reported upregulated LO expression in OSMF and OSCC. By examining oral biopsies from patients with OSMF, OSCC arising in OSMF, and OSCC unrelated to OSMF, LO co-localized with strongly stained collagen and elastin areas in histochemical stainings. SCC tissues revealed LO localization adjacent to invading epithelial islands, indicating stromal reactions in both OSMF-related and non-OSMF-related SCC cases. These findings suggest that LO upregulation may be vital in OSMF pathogenesis and early stromal reactions in oral cancer [52]. The high copper content in areca ingredients has been demonstrated [53, 54]. Elevated soluble copper levels found in oral fluids of chronic chewers further support the role of copper as an initiating factor in OSMF, stimulating fibrosis by upregulating LO activity [52, 53, 54]. Areca and copper can induce LO, and LO possesses transcription activator functions that can activate collagen III and elastin promoters in OSMF [52]. The substantial copper release from areca products is believed to induce LO activity, enhancing collagen synthesis by fibroblasts, promoting cross-linking, and inhibiting degradation [55, 56].
Cancer cells experience mechanical stress within their primary environments as well as during their migration through the bloodstream and various intravascular stages of the metastatic cascade [57]. As tumor cells circulate in the bloodstream or lymphatic vessels, they endure diverse mechanical stresses due to hemodynamic forces and vasoconstriction. Circulating tumor cells (CTCs) must withstand these stresses for the metastatic cascade to be successful (Fig. 1). High fluid shear stress levels can induce cell cycle arrest [58] and even lead to the destruction of circulating tumor cells [59]. Consequently, only a small fraction of CTCs can evade the effects of fluid shear stress and adhere to the endothelium. CTC clusters have been observed traversing capillary-sized vessels [60].
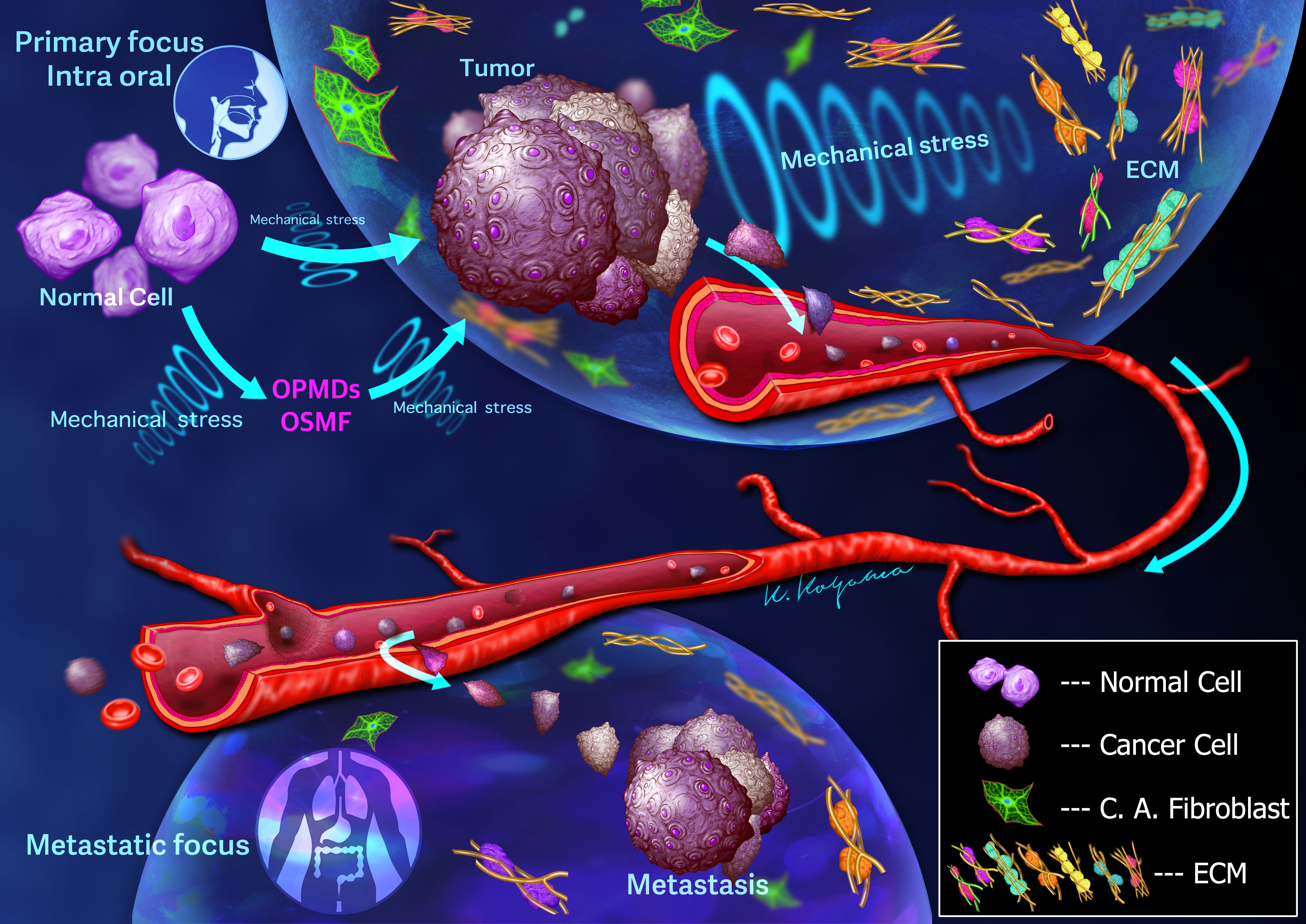
Schematic illustration depicts the invasion of cancer cells into the vasculature, where they may grow or detach as single cells or clumps and travel through the circulatory system. Flexible movement using blebs is necessary for invasion and metastasis of cancer cells. Both cancer cells and cancer-associated fibroblasts interact with the extracellular matrix (ECM) components, resulting in various biological processes that determine the fate of cancer. Furthermore, not only cancer cells but also normal cells are definitely affected by mechanical stress, leading to the development of oral potentially malignant disorders (OPMDs) and oral submucous fibrosis (OSMF).
Tumor stiffness is associated with increased interstitial tissue pressure and solid stress stemming from disrupted vasculature, tumor expansion [61], and fibrosis [62]. Solid cancer progression frequently accompanies an increase in matrix stiffness, primarily owing to the deposition of large amounts of ECM proteins such as collagen, fibronectin, or hyaluronic acid by activated stromal fibroblasts [63]. Paszek et al. [64] noted that matrix stiffness triggers intracellular signaling and malignant transformation. Briefly, matrix stiffness can potentiate tumor malignancy. Additionally, tumor stiffness can impact treatment effectiveness. Solid tumors often exhibit intrinsic resistance to treatment, partly attributed to the hindrance of blood-borne drug delivery to cancer cells [65, 66]. Tumor and stromal cells generate a matrix comprising collagens, proteoglycans, and other molecules that impede macromolecule transport. This matrix stiffness contributes to resistance. Netti et al. [67] demonstrated that collagen organization and the combination of collagen and proteoglycans significantly contribute to interstitial transport resistance in human colon adenocarcinoma, human glioblastoma, human soft tissue sarcoma, and murine mammary carcinoma. When hepatocellular carcinoma (HCC) cells were cultured in media with varying matrix stiffness, cells cultured in the stiffest medium exhibited the highest resistance to paclitaxel, 5-FU, and cisplatin. These findings indicate that matrix stiffness substantially influences the chemoresistance of HCC cells [68]. We propose that matrix stiffness constitutes an aspect of chemoresistance. Consequently, novel anticancer therapies, such as gene therapy and immunotherapy, might be ineffective owing to their status as high-molecular-weight drugs that cannot effectively permeate the tumor stroma.
Oral cancer, which originates from the mucosal epithelium of the oral cavity, represents the most prevalent form of head and neck cancer. The incidence of oral cancer varies across countries or regions and is typically associated with exposure to tobacco-derived carcinogens, excessive alcohol consumption, or a combination of both [69]. Notably, the de novo type characterized by induration highlights the impact of tumor invasion depth on metastasis and prognosis [70]. To advance oral cancer treatment, comprehending the complex interplay between genetic regulation, tissue stiffness, and environmental factors within the host environment is crucial. Functional analysis and tumor structure evaluation serve as vital tools in cancer treatment. The breakdown of the basement membrane, resulting from tissue force and protease enzyme digestion of the ECM, is responsible for the invasion of the mesenchyme and the formation of metastatic oral cancer cells. Carcinoma cells initiate the metastatic cascade and extend invasive pseudopodia through breaches in the basement membrane—an extracellular matrix macromolecular barrier that underlies epithelial cells and encapsulates blood vessels [71]. A subset of metalloproteinases from the matrix metalloproteinase gene family is consistently upregulated in invasive carcinomas [72, 73]. Cancer metastasis involves a sequence of steps, commencing with the invasion of the adjacent normal stroma. Upon penetration of vascular or lymphatic channels, invading cells may either grow, detach as individual cells or clusters, and navigate the circulatory system (Fig. 1). Cancer cells surviving the host’s immune defenses, non-immune defenses, and circulation turbulence subsequently arrest within the capillary bed of susceptible organs, undergo extravasation into organ parenchyma, propagate, and initiate metastasis [74].
The genesis of OSMF in a subset of areca chewers might be related to LO, a
copper-activated enzyme pivotal in collagen cross-linking and ECM organization
[75]. Betel quid (BQ) can impact ECM turnover by regulating factors like
TGF-
In this review, we have also discussed the regulation of mechanical stress and its signaling pathways in OSMF, OPMDs, and cancer cells. Nonetheless, comprehending the underlying mechanisms of each disease in distinct nationalities and populations remains essential. Alterations in tumor structure, particularly arising from cancer cell and matrix stiffness, offer predictive value for human cancer outcomes. Stiffness holds promise for prognosis prediction and may function as a therapeutic target. In the context of solid tumors, targeting such properties through drug intervention during induction chemotherapy to manage tumor hardness and its associated microenvironment, followed by assessing sensitivity, could prove effective. Alternatively, even with the same surgical procedure, a strategy involving the decision to perform a resection with a larger margin could be considered.
TH contributed to the research design and prepared the manuscript. TH listed previous reports for this review and critically revised the manuscript. TO contributed to the research design and conception of this work and oversaw. TO also prepared the manuscript. YS prepared the manuscript, interpreted the conception and critically revised the manuscript. SA contributed to the research design and prepared the manuscript. SA also listed previous reports for this review and critically revised the manuscript. TN contributed to the preparation of the manuscript. TN also interpreted the conception and critically revised the manuscript. All authors read and approved the final manuscript. All authors have participated sufficiently in the work and agreed to be accountable for all aspects of the work.
Not applicable.
We would like to thank Editage (https://www.editage.com) for the English language editing. We are grateful to the KAAK studio (Keisuke Koyama: Dentist and Oral Surgeon) for preparing the illustration.
This work was supported by grants-in-aid (Grant Numbers 22K17025 to (T.O.) and 22K10200 to (S.A.)) for Scientists’ Research from MEXT, Japan.
The authors declare no conflict of interest. Given his role as Guest Editor, Takehito Ouchi had no involvement in the peer-review of this article and has no access to information regarding its peer review. Full responsibility for the editorial process for this article was delegated to Amedeo Amedei.
Publisher’s Note: IMR Press stays neutral with regard to jurisdictional claims in published maps and institutional affiliations.