- Academic Editor
Background: The intensive and injudicious use of pesticides in
agriculture has emerged as a major concern due to its detrimental impact on
aquatic ecosystems. However, the specific impact of broad-spectrum fungicides,
such as Thiophanate-methyl (TM), on cyanobacteria remains a subject of ongoing
research and debate. Methods: In order to fill this knowledge gap, The
present study aimed to comprehensively investigate the toxicological effects of
TM (10–30 µg/L) on the growth, photosynthetic pigments, oxidative
stress, and biochemical composition of the non-nitrogen-fixing cyanobacterium
Arthrospira platensis. Results: Our findings unequivocally
demonstrated that TM exposure significantly inhibited the growth of A.
platensis. Moreover, the decrease in chlorophyll content indicated a pronounced
negative impact on the photosynthetic system of A. platensis caused by
TM exposure. Notably, TM induced oxidative stress in A. platensis, as
substantiated by a significant increase in lipid peroxidation (MDA) within the
culture. Furthermore, the intracellular generation of hydrogen peroxide
(H
In agriculture worldwide, fungicides are extensively employed to control the proliferation of unwanted noxious plants. However, the use of these pesticides in large quantities raises significant concerns due to their potential toxicity. When used excessively, fungicides can have detrimental effects on agricultural soil, plants, aquatic life, and human organisms. Among the biocides utilized to inhibit the growth of fungi, bacteria, and parasites in crops, animals, and humans, the benzimidazole fungicides group is widely prevalent [1]. One specific member of this group is Thiophanate methyl (TM), which serves as a systemic fungicide extensively utilized in agriculture to protect crops against fungal diseases [2]. TM is classified as a grade IV or low-toxicity chemical, indicating a relatively lower risk to human health and the environment. However, even though it falls into this category, TM can still be detected in soil samples as well as in vegetables and fruits, classifying it as a “pesticide residue” of growing concern. Consequently, there is an increasing need to investigate the potential ecological and health impacts associated with the presence of TM residues [3]. Benzimidazole fungicides harmfully affect soil microbial activity, such as rhizosphere microbes diversity, abundance and composition , all of which impact microbial mineralization of organic molecules, nutrient cycling and bioavailability, pollutants detoxification, and xenobiotic bioremediation, leading to soil degradation and yield loss [4, 5]. Furthermore, TM can enter aquatic ecosystems through water runoff, posing a serious threat to freshwater microalgae. These microorganisms play a vital role in the ecosystem as they contribute to oxygen production and act as a crucial food source for other organisms. Therefore, the pollution of aquatic environments with TM can disrupt the balance of the ecosystem and have far-reaching consequences [3]. Studies have revealed the adverse effects of TM exposure on various organisms. For instance, TM has been found to induce developmental and notochord toxicity in zebrafish (Danio rerio) embryos [6]. This highlights the potential risks associated with TM exposure, even at early stages of development. However, the specific toxicological effects of TM on Spirulina platensis, a significant prokaryotic microalgae species, remain largely unexplored [6].
Photoautotrophic organisms such as green algae and cyanobacteria are the base of the food web in aquatic ecosystem. They provide a large amount of oxygen and food for other organisms and affect the stabilization of the hole ecological system [7]. Moreover, they play an essential role in minerals cycling. Spirulina platensis, a member of the cyanobacteria phylum, stands out as a significant prokaryotic microalgae species among several others. Its extensive usage by humans over an extended period of time can be attributed to its remarkable nutritional value. With protein constituting around 60% of its dry weight, Spirulina platensis is rich in essential components like amino acids, vitamins, minerals, and unsaturated fatty acids [8]. Recently, it was found that Arthrospira platensis (formerly known as Spirulina platensis) was very sensitive to the environmental pollutants [9]. For example, research conducted by Jiang et al. [10] demonstrated the toxicological effects of antibiotics norfloxacin (NFX) and sulfadiazine (SDZ) on Arthrospira platensis. Furthermore, a study by Abbasi et al. [11] revealed that polyethylene microplastics induced adverse effects on the growth and photosynthetic activity of Spirulina. These effects included oxidative stress, fragmentation, and swelling of trichomes. Such findings emphasize the importance of studying the influence of pesticides and other emerging pollutants on microalgal growth and population dynamics. By gaining insights into the ecotoxicological risks associated with these substances, it becomes possible to assess and mitigate their potential harm to ecosystems [11]. Furthermore, the toxicological effect of TM on the Spirulina platensis remains unclear.
In the present study, our main objective was to investigate the intricate relationship between pesticide residues, specifically the fungicide TM, and their impact on aquatic ecosystems. We focused on examining the influence of different dosages of TM on various physiological parameters using Arthrospira platensis as the model organism. This choice was based on the significance of Arthrospira platensis in understanding the response of primary producers in aquatic environments. TM is a commonly detected pesticide residue in aquatic ecosystems, making it a critical area of research due to its potential ecological hazards. Our study aimed to provide comprehensive insights into the impact of TM and other pesticide residues on the delicate balance of aquatic ecosystems. By specifically exploring the effects of TM on Arthrospira platensis, we aimed to shed light on the potential risks and threats posed by these residues to the stability and health of aquatic environments. Understanding the ecological implications of pesticide residues, including TM, is of paramount importance due to their persistence in water bodies and far-reaching consequences for the organisms inhabiting these ecosystems. Our research contributes to the growing body of knowledge concerning pesticide residue pollution in aquatic ecosystems. It also highlights the urgent need to adopt sustainable and environmentally-friendly agricultural practices to mitigate the risks associated with pesticide residues. Through a comprehensive analysis of various physiological parameters of Arthrospira platensis, such as growth, photosynthetic pigments, oxidative stress, and biochemical composition, we aimed to unravel the complex interactions between pesticide residues and microalgae. This knowledge is essential for developing effective strategies to mitigate the adverse effects of pesticide residues on aquatic ecosystems. Ultimately, our study seeks to ensure the long-term sustainability and health of our water resources.
This work represents a novel contribution as it explores a research area that has not been previously investigated. The specific investigation of the influence of different dosages of the fungicide TM on various physiological parameters of Arthrospira platensis in the context of aquatic ecosystems is a unique aspect of this study. By examining its growth, photosynthetic pigments, oxidative stress, and biochemical composition, the study offers a detailed understanding of how pesticide residues, including TM, affect this microalgae. By providing detailed information on the specific effects of TM on Arthrospira platensis, the study enables a more accurate assessment of the associated risks. It also emphasizes the need for effective environmental management measures to mitigate these risks. Lastly, the findings of this study have important implications for sustainable management practices. By highlighting the significance of environmentally friendly agricultural practices, the article underscores the importance of reducing risks associated with pesticide residues to preserve the long-term health and sustainability of aquatic ecosystems.
Commercial formulation of Thiophanate-methyl (70% waters dispersible granule) as active ingredient, was used as test substance in the study. It is purchased from local market of Medea, Algeria. The selection of fungicide was based on a survey conducted in Medea province in 2022, which indicated that Thiophanate-methyl was the most widely used active ingredient pesticides in the region. All other reagents and solvents used were of analytical grade.
In this study, the selection of cyanobacteria Arthrospira platensis as
the test species holds significant importance. This particular strain of
cyanobacteria was collected from a sebkha located in the Sahara region of
Tamanrasset, Algeria, representing a unique ecological niche. By using this
specific strain, the study aims to capture the responses and adaptations of
Arthrospira platensis to the fungicide TM under controlled laboratory
conditions. To facilitate the cultivation of Spirulina, 500 mL
Erlenmeyer flasks were utilized, each containing sterilized Zarrouk culture
medium. The pH of the culture medium was carefully adjusted to 9.1
Throughout the experimental period, the cultures were maintained at a constant
temperature of 34
In order to accurately assess the biomass of Arthrospira platensis, the dry cell weight (DCW) was utilized as a quantitative measure. The DCW serves as a reliable indicator of the cellular mass of the cyanobacterial culture and is commonly used in such studies. To determine the DCW, optical density measurements at a specific wavelength were employed.
In this study, the optical density of the cultures was measured at 618 nm, a wavelength commonly used for assessing the growth and biomass of Arthrospira platensis. The optical density is proportional to the concentration of cells in the culture, allowing for the estimation of biomass. To convert the measured absorbance values to dry weight, a conversion equation was applied. This equation takes into account the relationship between the optical density and the actual dry weight of the cells. The specific conversion equation used in this study was determined based on previous calibration experiments and validation studies. By applying following equation, the optical density measurements obtained at 618 nm were transformed into corresponding dry weights.
The impact of different dosages of the fungicide TM (ranging from 0 to 30 µg/L) on the growth and physiological parameters of Arthrospira platensis was assessed using the IR. The IR provides a quantitative measure of the extent to which TM inhibits or affects the biological processes of the cyanobacterium [13].
To calculate the IR, the following formula was utilized:
IR (%) = (1 – T/C)
Where:
IR = Inhibition Rate
C = Control Value (physiological parameter without TM exposure)
T = Test Value (physiological parameter with TM exposure)
In this study, various physiological parameters of A. platensis were assessed, such as growth rate, photosynthetic pigments, oxidative stress markers, and biochemical composition. The IR was calculated for each parameter at different TM dosages. The IR values indicate the degree of inhibition or alteration of each physiological parameter caused by TM exposure. A higher IR signifies a greater impact of TM on the specific parameter, indicating increased sensitivity or susceptibility of A. platensis to the fungicide.
To determine the content of chlorophyll a, chlorophyll b, and carotenoids in Arthrospira platensis, a solvent extraction method using 3 mL of 96% ethanol was employed. The extraction process allowed for the release and solubilization of these photosynthetic pigments present in the cyanobacterial cells. Following the extraction, the concentrations of chlorophyll a, chlorophyll b, and carotenoids were quantified using spectrophotometric measurements at specific wavelengths. The absorbance of the extracted pigment solution was measured at 665 nm for chlorophyll a, 649 nm for chlorophyll b, and 470 nm for carotenoids. To calculate the concentration of photosynthetic pigments, a mathematical equation previously described by a relevant source [14] was applied. This equation takes into account the absorbance values obtained from the spectrophotometer and allows for the determination of the pigment concentrations in the samples. By utilizing this equation, the researchers were able to accurately quantify the content of chlorophyll a, chlorophyll b, and carotenoids in Arthrospira platensis cultures exposed to different TM dosages. This provided valuable information on the impact of TM on the photosynthetic apparatus and pigment composition of the cyanobacterium.
V
A
Chl
Malondialdehyde (MDA) is a well-known biomarker used to assess lipid peroxidation, which is indicative of oxidative stress in cells. In this study, the content of MDA in A. platensis was determined as a measure of lipid peroxidation caused by TM exposure. To quantify the MDA content, a method described by a relevant source [15] was followed. The procedure involves the reaction of MDA with thiobarbituric acid (TBA) to form a colored complex, which can be measured spectrophotometrically. The MDA content was then expressed as micromoles per gram of fresh weight (µmol/g FW), providing a quantitative measurement of lipid peroxidation in the cyanobacterial cells.
Another parameter assessed to evaluate the oxidative stress induced by TM
exposure was hydrogen peroxide (H
To obtain the cell lysate for antioxidant and detoxification enzyme assays in A. platensis, a series of steps were followed. Initially, aliquots of the microalgae suspension, approximately 10 mL in volume, were collected. These aliquots were then subjected to centrifugation at 4 °C for 15 minutes at 15,000 rpm. The centrifugation step aimed to separate the microalgal pellets from the suspension. Following centrifugation, the microalgal pellets were washed multiple times with distilled water to remove any impurities or contaminants. The washing process involved repeated cycles of centrifugation and resuspension. After the final wash, the microalgal pellets were resuspended in 1 mL of 0.1 M Tris-HCl buffer with a pH of 7.4. To disrupt the microalgal cells and release the cellular contents, the resuspended microalgal pellets were subjected to sonication. The sonication process was carried out at 4 °C for 5 minutes. Subsequently, the sonicated samples were centrifuged at 4 °C for 10 minutes at 15,000 rpm to separate the cell debris from the cell lysate.The supernatant obtained after centrifugation, which contained the cell lysate, was collected for further analysis of antioxidant and detoxification enzyme activities [14]. The specific enzymes measured in this study included catalase (CAT), ascorbate peroxidase (APX), glutathione-S-transferase (GST), and peroxidase (POD). The assay methods for these enzymes were performed according to previously described protocols [17, 18, 19, 20], respectively.
The enzyme activities were quantified and expressed as units per milligram of protein (U/mg protein), providing a measure of the enzyme’s catalytic efficiency. These enzyme activities serve as indicators of the antioxidant and detoxification capacities of A. platensis in response to TM exposure.
To assess the concentration of free carbohydrates in the biomass of A.
platensis, the Anthrone method was employed [21]. Glucose was used as the
standard for calibration. The biomass, obtained from 5 mL of the culture, was
subjected to centrifugation at 15,000
For the determination of lipid concentration, the sulfo-phospho-vanillin method, as described by Mishra et al. [22] was employed In this method, a commercial canola oil was used as the standard. The biomass collected from the culture was subjected to the same centrifugation process as mentioned earlier. The resulting cell pellet was mixed with the appropriate reagents according to the sulfo-phospho-vanillin method, and the lipid concentration was determined.
To measure the protein content in the biomass, the bovine serum albumin standard method, as previously described by Bradford et al. [23], was utilized. The biomass was processed using the same centrifugation procedure mentioned above. The resulting cell pellet was subjected to protein extraction and quantification using bovine serum albumin as the standard.
The data presented in this study are expressed as mean
The exposure to TM had a significant (p
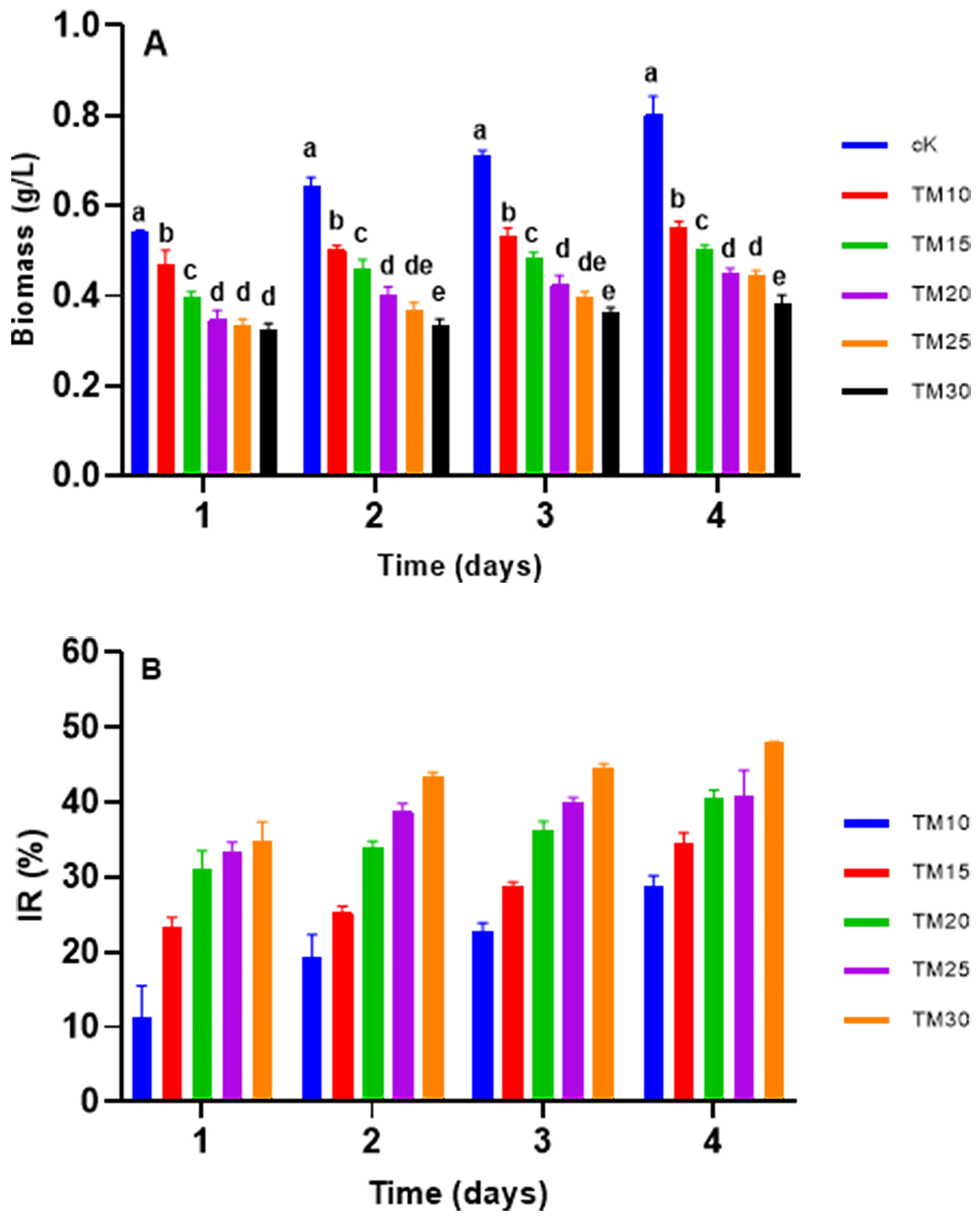
Effect of thiophanate-methyl (TM) on
Arthrospira platensis growth in terms of biomass (A) and specific growth
inhibition rate (IR) (B) within 4 days of cultivation. Error bars indicate
standard deviation (n = 3). Significant differences obtained statistically were
indicated by different letters and styles (p
The IR of A. platensis, which reflects the extent of growth suppression caused by TM, followed a similar trend as the biomass measurements. The IR increased gradually with higher TM concentrations, indicating a dose-dependent response (Fig. 1B). After 4 days of exposure, the IR of A. platensis was enhanced by 29%, 35%, 40%, 41%, and 48% in the presence of TM10, TM15, TM20, TM25, and TM30, respectively (Fig. 1B).
These findings highlight the sensitivity of A. platensis to TM exposure, suggesting that this fungicide can significantly impair the growth and development of this cyanobacterial species. The dose-dependent relationship observed between TM concentration and growth inhibition underscores the importance of considering the potential ecological risks associated with the use of this pesticide in aquatic environments. Further research is needed to elucidate the underlying mechanisms and specific biochemical pathways involved in the inhibitory effects of TM on A. platensis. Such knowledge is crucial for developing effective strategies to mitigate the detrimental impacts of pesticide residues and safeguard the health and balance of aquatic ecosystems.
The observed changes in pigment contents of A. platensis under the influence of TM provide valuable insights into the physiological response of this cyanobacterium to fungicide exposure. The significant decrease in chlorophyll a and b contents suggests an impairment in the photosynthetic system of A. platensis caused by TM exposure (Fig. 2). Chlorophylls are crucial pigments involved in capturing light energy for photosynthesis, and their reduction indicates a disturbance in the photosynthetic process. The increase in carotenoid contents (Fig. 2), on the other hand, can be seen as a protective response of A. platensis to TM-induced stress. Carotenoids play a vital role in photoprotection by dissipating excess light energy and scavenging reactive oxygen species. The elevated carotenoid levels observed in this study suggest that A. platensis activates its defense mechanisms to mitigate the oxidative stress caused by TM exposure.
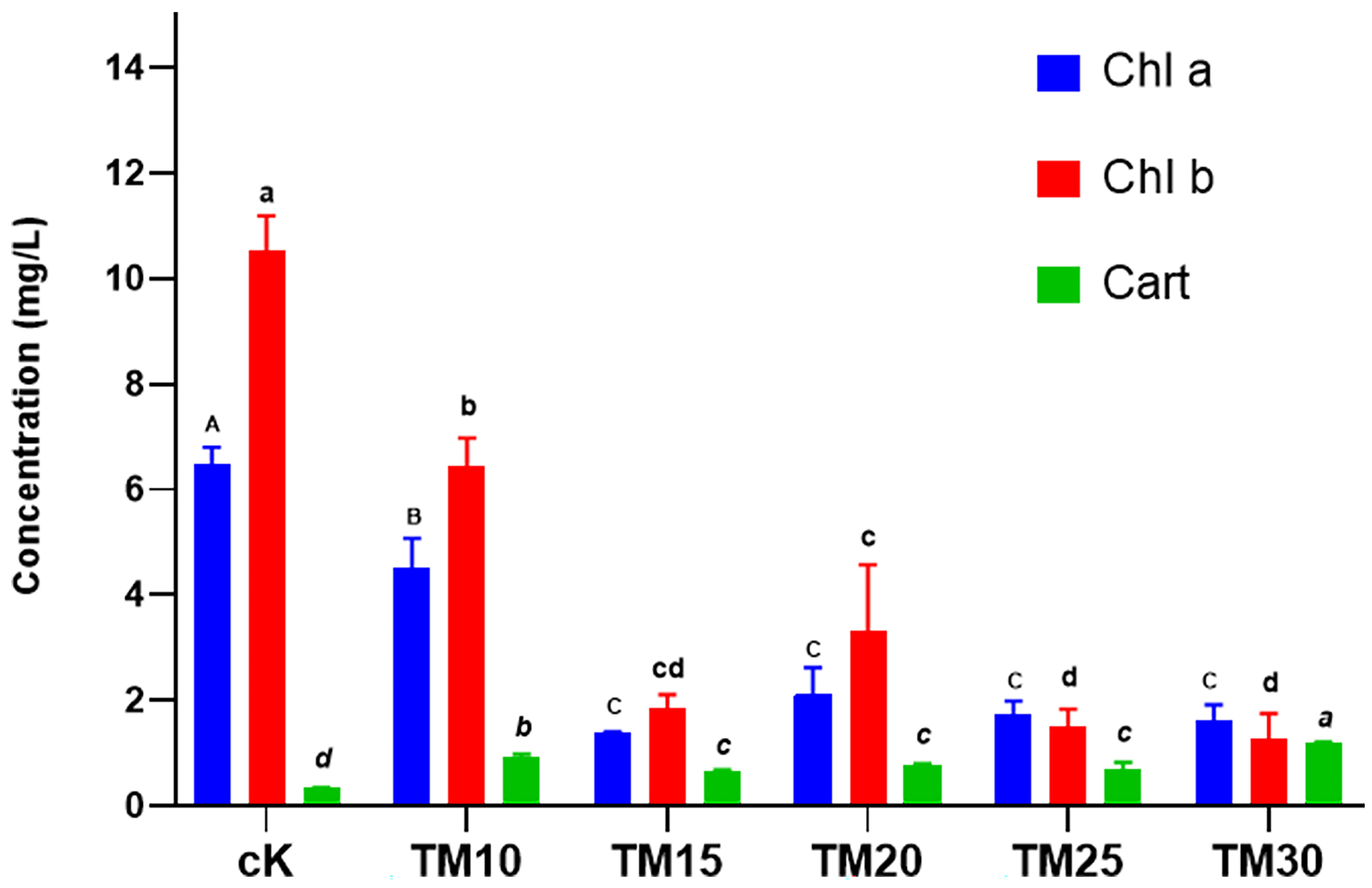
Effect of TM on the contents of chlorophyll a, b and
carotenoids in Arthrospira platensis. Error bars indicate standard
deviation (n = 3). Significant differences obtained statistically were indicated
by different letters and styles (p
The contrasting responses of chlorophylls and carotenoids to TM exposure highlight the complexity of the physiological adjustments occurring in A. platensis. These findings indicate that TM not only disrupts the photosynthetic machinery but also triggers adaptive responses aimed at maintaining cellular homeostasis and survival. Understanding the changes in pigment composition provides important insights into the overall health and functioning of A. platensis in response to environmental stressors like TM. These pigment-based responses can serve as indicators of the ecological health of aquatic ecosystems, as cyanobacteria are primary producers that play a crucial role in nutrient cycling and food webs.
In Fig. 3, the results illustrate the significant impact of TM fungicide on the
induction of MDA and H
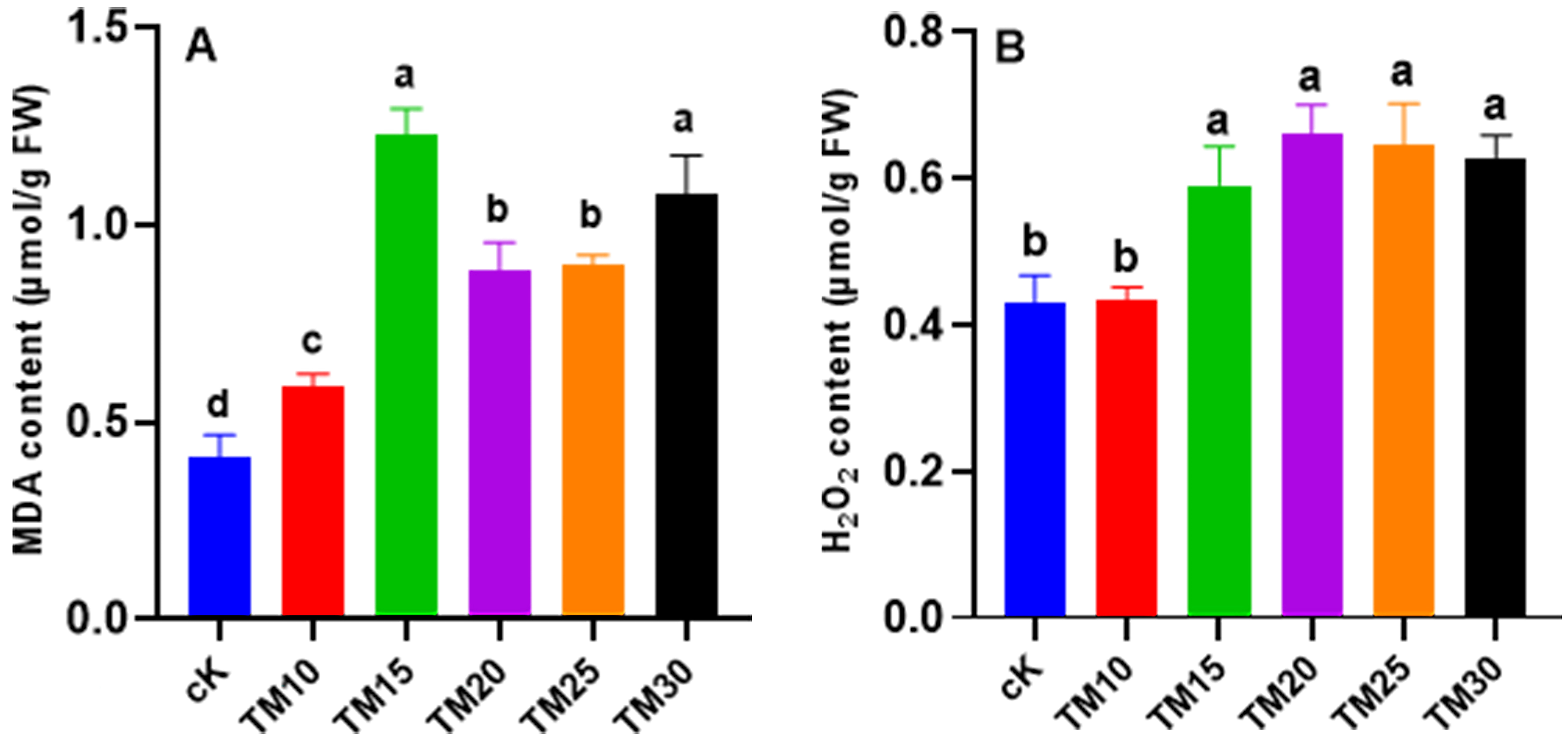
Effect of TM on the contents of MDA (Malondialdehyde) (A) and
H
In Fig. 4, the activity of APX in A. platensis
demonstrated a significant difference (p
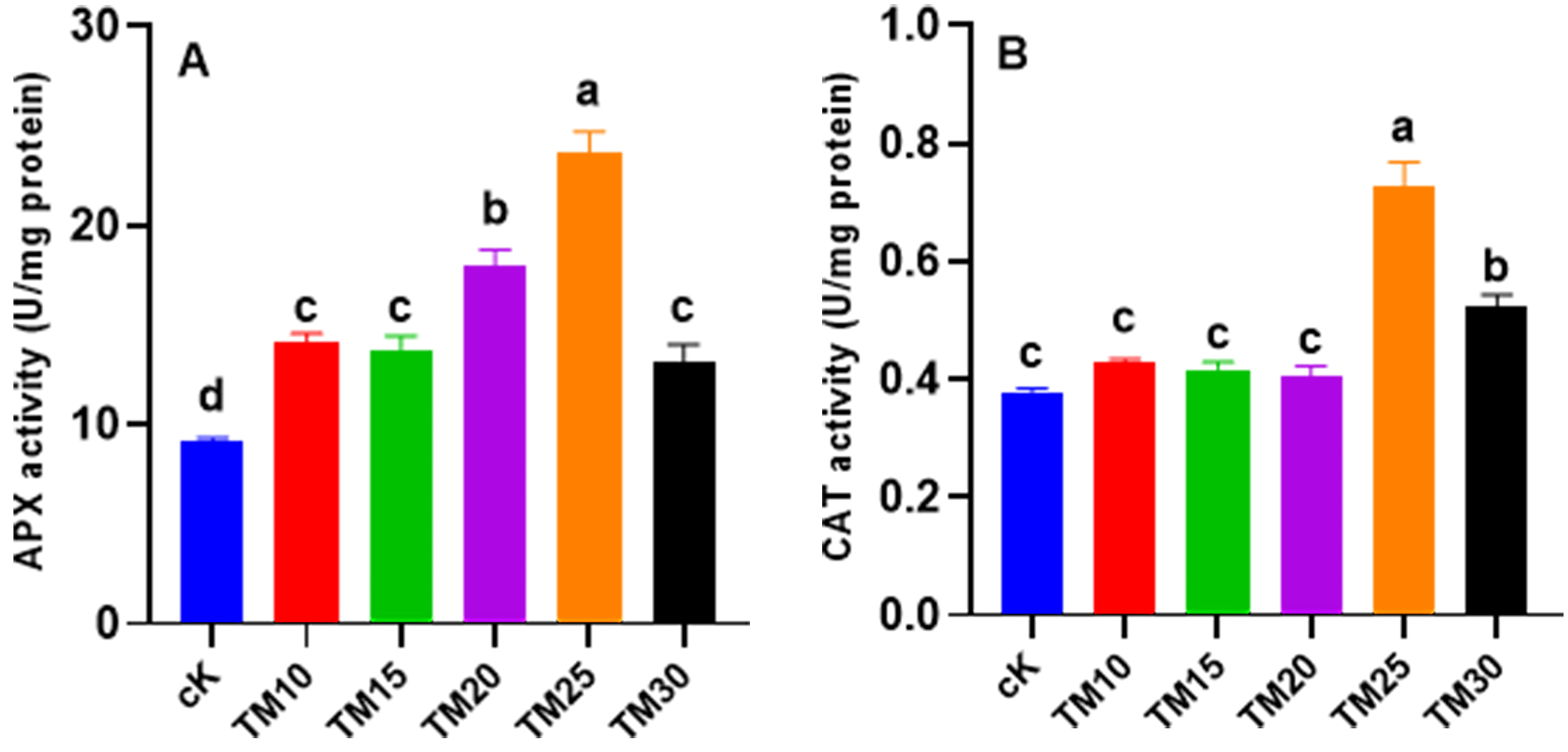
Effect of TM on the activity of APX (ascorbate peroxidase) (A) and CAT (catalase) (B) in
Arthrospira platensis. Error bars indicate standard deviation (n = 3).
Significant differences obtained statistically were indicated by different
letters (p
In Fig. 5, the activity of GST in A.
platensis was significantly (p
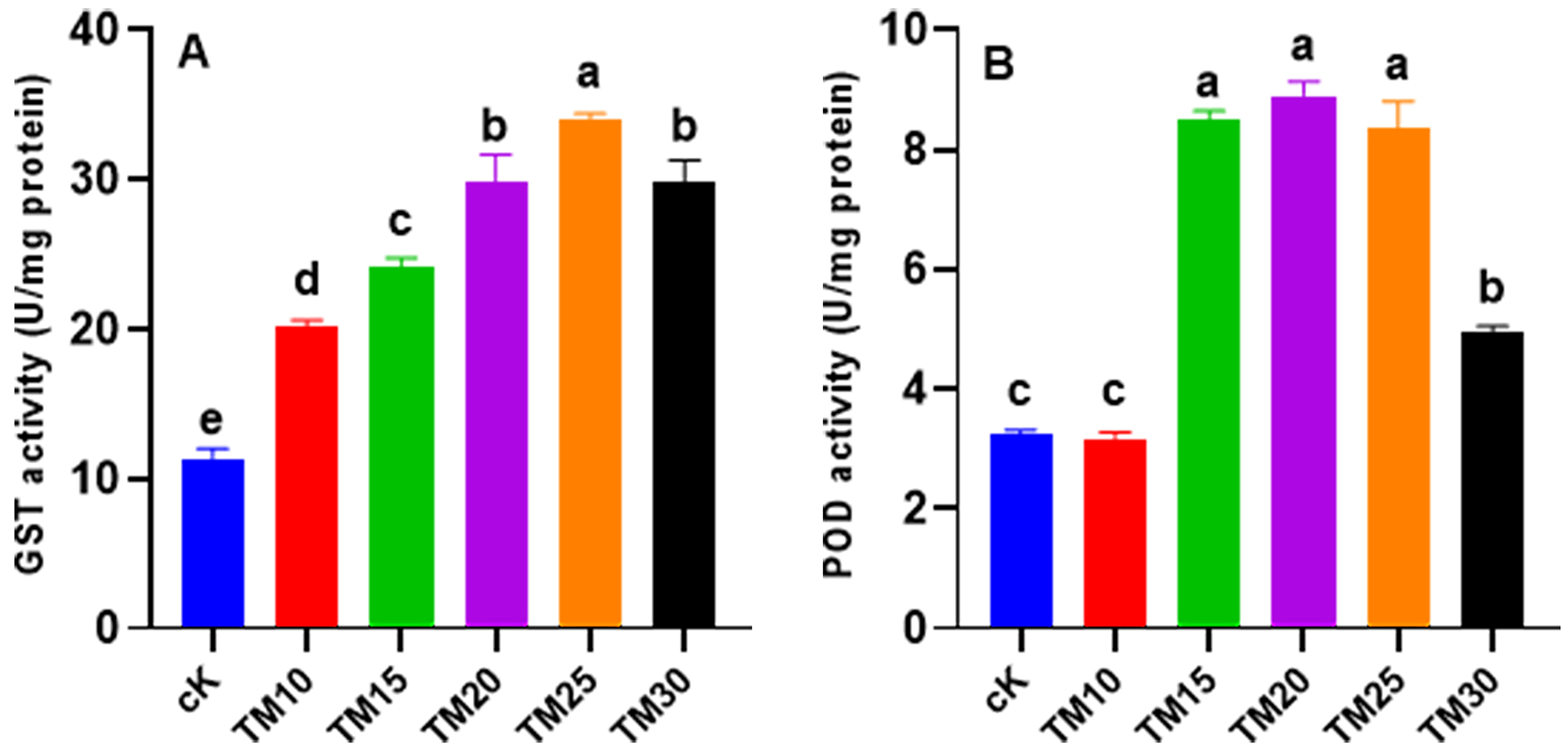
Effect of TM on the activity of GST (glutathione-S-transferase) (A) and POD (peroxidase) (B) in
Arthrospira platensis. Error bars indicate standard deviation (n = 3).
Significant differences obtained statistically were indicated by different
letters (p
In Fig. 6, the effects of TM fungicide on the levels of various bio-components
in A. platensis are presented. Exposure to TM at concentrations ranging
from 10 to 30 µg/L resulted in significant (p
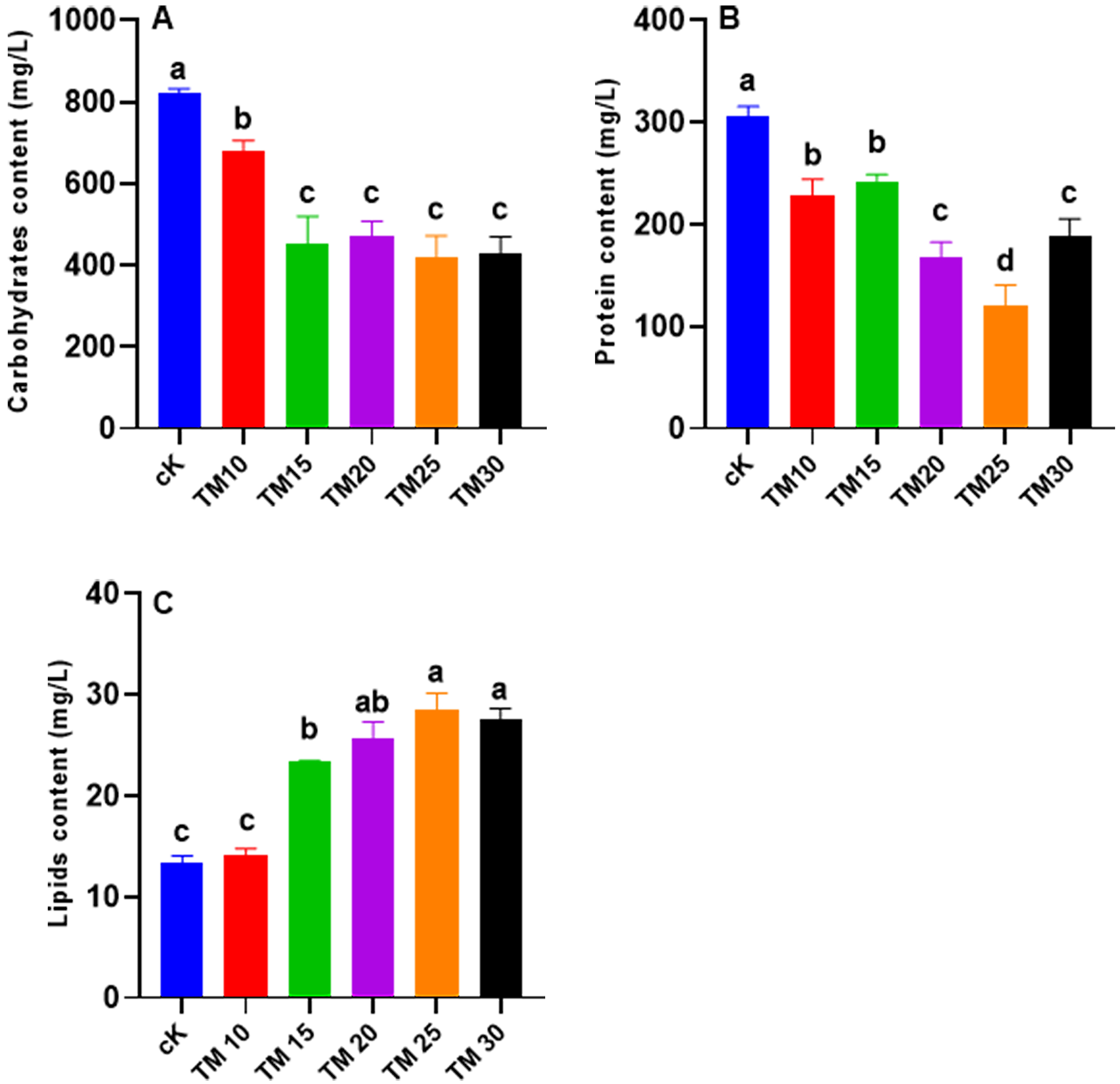
Effect of TM on the contents of carbohydrates (A),
proteins (B) and lipids (C) in Arthrospira platensis. Error bars
indicate standard deviation (n = 3). Significant differences obtained
statistically were indicated by different letters (p
Several studies have investigated the effects of pesticides on freshwater microalgae, highlighting the potential ecological risks associated with these chemical contaminants [24, 25, 26]. However, limited research has been conducted on the specific impact of fungicides on cyanobacteria, such as Arthrospira platensis. In this study, we aimed to fill this knowledge gap by investigating the effects of TM, a common fungicide found in aquatic ecosystems, on the growth, biocomponents, and oxidative stress responses of A. platensis. Our findings revealed a clear and significant impact of TM exposure on the biomass of A. platensis, indicating a direct inhibition of growth (Fig. 1A). Interestingly, this growth inhibition exhibited a dose-dependent relationship with TM concentrations and was also influenced by the duration of exposure (Fig. 1B). These results are consistent with previous studies that have reported similar inhibitory effects of pesticides on microalgae [27]. For instance, studies have demonstrated the high toxicity of glyphosate to cyanobacteria such as Pseudanabaena sp. [28]. Furthermore, recent research has highlighted the negative effects of microplastics, particularly polyethylene, on the growth of spirulina [11]. The observed growth inhibition can be attributed to the excessive production of ROS as a result of membrane lipid damage caused by xenobiotic compounds [29]. This oxidative stress disrupts essential processes such as chlorophyll synthesis, ultimately leading to decreased biomass production. Additionally, we observed that the IR increased with prolonged exposure and higher concentrations of TM fungicide. Notably, the highest IR was observed after 96 hours of TM30 treatment, indicating a more pronounced growth inhibition effect at higher concentrations (Fig. 1B). These findings are consistent with a study conducted by Dong et al. [13], which demonstrated similar concentration-dependent effects of sulfamethoxazole (SMZ) on Chlorella vulgaris and Microcystis aeruginosa.
Chlorophyll pigments are crucial for the process of photosynthesis in microalgae [30]. Chlorophyll a, in particular, plays a key role in capturing light energy and converting it into chemical energy [31]. The significant decrease in chlorophyll a and b contents observed in A. platensis exposed to TM fungicide indicates a disturbance in the photosynthetic capacity of the microalgae (Fig. 2). This reduction in chlorophyll levels can be attributed to the negative effects of TM on the photosynthetic apparatus, such as damage to the electron transfer system of PSII and the accumulation of ROS [32]. These findings align with previous studies that have reported decreases in chlorophyll content in microalgae exposed to different pollutants [10, 14, 33]. Furthermore, the positive correlation between chlorophyll a content and biomass highlights the close relationship between photosynthetic activity and the growth of A. platensis (Figs. 1 and 2). The decline in chlorophyll pigments due to TM exposure can have a detrimental impact on the overall productivity and biomass accumulation of the cyanobacteria. This suggests that TM fungicide poses a potential hazard to the growth and development of A. platensis. On the other hand, carotenoids play a protective role in microalgae by absorbing excessive light energy and quenching ROS, thereby preventing oxidative damage to the photosynthetic apparatus [31]. In our study, the increased content of carotenoids in response to TM exposure indicates a compensatory mechanism employed by A. platensis to counteract the harmful effects of the fungicide (Fig. 2). Carotenoids act as antioxidants and help maintain the stability of photosynthetic performance, protecting the microalgae from oxidative stress caused by TM exposure. These findings are consistent with previous research that has demonstrated the ability of certain contaminants to enhance carotenoid levels in microalgae, thus improving their antioxidant capacities [14]. Overall, the significant decrease in chlorophyll content and the concurrent increase in carotenoid content highlight the adverse effects of TM fungicide on the photosynthetic activity of A. platensis. These findings underscore the importance of assessing the impacts of environmental contaminants on microalgae, as they serve as vital components of aquatic ecosystems and play a significant role in global biogeochemical cycles [34]. Understanding the responses of microalgae to contaminants is crucial for evaluating the ecological implications and developing strategies for environmental management and conservation.
Exposure to aquatic xenobiotics can disrupt the balance of ROS in freshwater microalgae, resulting in oxidative stress [32]. A byproduct of lipid peroxidation is commonly used as a biomarker to evaluate the degree of oxidative stress induced by pollutants such as fungicides [35]. In our study, we observed a significant increase in MDA content in A. platensis (Fig. 3A), indicating that TM stress adversely affected the integrity of cell membranes and led to oxidative damage to unsaturated fatty acids [14]. The elevated MDA levels observed can be attributed to the excessive generation of ROS following exposure to TM fungicide (Fig. 3B). These findings align with a previous study that reported increased MDA contents in the cyanobacterium Nostoc muscorum under captan fungicide stress [35]. The measurement of MDA serves as a valuable tool for assessing oxidative stress and lipid peroxidation in microalgae exposed to xenobiotics. Increased MDA levels indicate the occurrence of oxidative damage and disruption of membrane integrity, which can have significant implications for the overall health and physiological functions of microalgae. By quantifying MDA, we gain insights into the extent of oxidative stress induced by TM fungicide in A. platensis, shedding light on the potential risks posed by this pollutant to aquatic ecosystems. It is important to recognize that the generation of ROS and subsequent oxidative stress are pivotal mechanisms underlying the toxic effects of xenobiotics on microalgae. Oxidative stress can lead to cellular damage, impaired growth, and reduced photosynthetic efficiency, ultimately impacting the overall health and survival of microalgae populations. Monitoring and understanding the oxidative stress response in microalgae are crucial for assessing the ecological impacts of pollutants and developing strategies to mitigate their adverse effects on aquatic environments.
Cyanobacteria, including Spirulina,
have evolved a sophisticated enzymatic antioxidant defense system to counteract
the oxidative damage induced by pollutants [13, 32]. One of the key antioxidant
enzymes in this system is catalase (CAT), which plays a vital role in converting
H
GST is a crucial enzyme involved in the detoxification of xenobiotics by catalyzing the conjugation reaction with glutathione (GSH) in phase II of xenobiotic metabolism [33]. In our study, we observed that the catalytic activity of GST in A. platensis was significantly stimulated with increasing TM dosage (Fig. 5A). This dosage-dependent trend in the detoxification process coincided with the IR observed for the respective TM dosages (Fig. 1B). These findings indicate that higher TM dosages had a greater impact and induced a stronger detoxification response in A. platensis. Moreover, our results provide evidence that fungicide pollution poses significant challenges to cyanobacteria, which respond by metabolizing the contaminants through phase II conjugation with GSH [35]. These findings are consistent with the study conducted by Hamed et al. [38], where greater catalytic activities of GST were observed in Scenedesmus obliquus and Nostoc muscorum under propiconazole treatment, supporting our present data (Fig. 5A). In addition to GST, we observed an induction of POD activity in A. platensis following exposure to TM, showing dose-dependent responses under fungicide stress (Fig. 5B). The enhanced POD activity due to TM exposure might play a vital role in the transformation of xenobiotics [38]. Several studies have investigated the impact of pesticides on cyanobacterial detoxification enzymes, revealing their involvement in the pathways of xenobiotic detoxification and elimination. For instance, Yisa et al. [39] found that the catalytic activity of GST and POD in Microcystis was promoted by the amoxicillin antibiotic, indicating their participation in xenobiotic detoxification processes.
Biochemical macromolecules, including carbohydrates, lipids, and proteins, play crucial roles in the adaptation of organisms to environmental stressors, such as pollutants [14, 40]. In our study, we observed significant changes in the contents of carbohydrates, proteins, and lipids in A. platensis following exposure to the fungicide TM (Fig. 6A,B and C). These alterations in macromolecule levels provide insights into the adaptive responses of cyanobacteria to xenobiotic stress. The decrease in protein content observed in A. platensis can be attributed to the catabolism of proteins into amino acids, which serve as an energy source to combat the adverse effects of fungicide stress [40]. Similarly, the reduction in carbohydrate content suggests a disruption in carbohydrate synthesis pathways, potentially affecting the energy metabolism of cyanobacteria (Fig. 6A). This finding aligns with previous studies that reported decreases in protein and carbohydrate contents in spirulina exposed to antibiotic treatments [10]. In contrast to proteins and carbohydrates, the lipid content in A. platensis showed an increase following TM exposure (Fig. 6C). This elevation in lipid accumulation can be linked to the enhanced generation of ROS triggered by TM exposure (Fig. 3B). Cyanobacteria may utilize lipids as a defense mechanism, as lipids can absorb organic xenobiotics and restrict their bioavailability, thereby mitigating their toxic effects [10, 40]. This phenomenon has also been observed in other studies, such as the significant lipid accumulation in Chlorella sp. exposed to the insecticide alpha-cypermethrin [41]. Moreover, the changes in lipid content reflect the modulation of lipid metabolism in response to membrane damage caused by fungicide exposure (Fig. 3A). The promotion of lipid metabolism supports membrane repair processes and helps maintain the integrity and functionality of the cell [10]. The disturbances in carbohydrate, protein, and lipid contents in A. platensis indicate the disruption of energy reserve synthesis and metabolic processes caused by fungicide exposure [42]. These alterations are indicative of the cyanobacteria’s attempts to cope with xenobiotic-induced stress. However, further investigations are warranted to elucidate the underlying mechanisms involved in the adaptive responses of cyanobacteria to environmental pollutants.
Overall, this study provides valuable insights into the effects of the fungicide TM on the growth, biocomponents, and oxidative stress responses of the cyanobacterium Arthrospira platensis. The results demonstrate a clear and significant inhibition of growth, which is dose-dependent and influenced by the duration of exposure. The decrease in chlorophyll content and the concurrent increase in carotenoid content indicate disturbances in the photosynthetic capacity of A. platensis and highlight the potential hazards posed by TM fungicide. Additionally, the elevation of MDA content suggests oxidative damage and disruption of membrane integrity, emphasizing the role of oxidative stress in the toxic effects of TM. The upregulation of antioxidant enzyme activities, such as catalase, ascorbate peroxidase, and glutathione-S-transferase, indicates the activation of adaptive defense mechanisms against oxidative stress and xenobiotic detoxification. Furthermore, the changes in carbohydrate, protein, and lipid contents reflect the adaptive responses of A. platensis to fungicide-induced stress, involving energy reserve utilization and modulation of lipid metabolism. These findings contribute to the understanding of the ecological risks associated with fungicide pollution and the adaptive mechanisms of cyanobacteria in response to environmental contaminants. It is crucial to assess the impacts of such pollutants on microalgae, considering their significance in aquatic ecosystems and global biogeochemical cycles. The evaluation of oxidative stress responses and the activity of detoxification enzymes in microalgae are essential for the assessment and management of environmental pollutants. Further research is needed to unravel the underlying mechanisms and pathways involved in the adaptive responses of cyanobacteria to fungicides and other xenobiotic compounds, facilitating the development of effective strategies for environmental conservation and management.
In the present study, TM fungicide was found to have significant and detrimental effects on Arthrospira platensis, a cyanobacterium commonly known as spirulina. One of the key findings of our study is the generation of ROS and lipid peroxidation in A. platensis following TM exposure. The observed lipid peroxidation indicates a disruption of the cell membrane’s integrity, which can have severe consequences for the overall health and functionality of the cyanobacteria. Furthermore, TM exposure led to alterations in the antioxidant and detoxification enzymatic responses of A. platensis. Another significant impact of TM on A. platensis is the reduction in pigment content, particularly chlorophyll. Additionally, our study revealed changes in the macromolecule contents of A. platensis following TM exposure. Specifically, carbohydrates and proteins showed a significant decrease, while lipid accumulation increased. These alterations in macromolecule levels reflect the adaptive adjustments made by Spirulina in response to TM-induced stress. To gain a deeper understanding of the molecular mechanisms underlying the observed effects, future studies employing transcriptomic and metabolomic approaches are warranted. This article contributes significantly by delving into the intricate relationship between pesticide residues, particularly TM, and Arthrospira platensis in aquatic ecosystems. It provides valuable insights into the potential ecological hazards posed by these residues and emphasizes the urgency of adopting sustainable agricultural practices to safeguard the health of these precious aquatic environments.
The data and material for the current study are included in this published article. There is no other data generated nor used. The datasets used and/or analyzed during the present experiments are available from the corresponding author on reasonable request.
NT, MA, CB, RA, AD, ZB, HT, JZ, AA: Performed experimental work. AA, NT, AHHB and HT: Supervision, Conceptualization, Writing — original draft. AA, JZ and MA: Co-Supervision. NT, AHHB, HT, JZ, and AA: Writing — review & editing. NT: Data Analysis. NT reviews excel and graphs. All authors read and approved the final manuscript. All authors have participated sufficiently in the work and agreed to be accountable for all aspects of the work. All authors contributed to editorial changes in the manuscript.
Not applicable.
Not applicable.
This research received no external funding.
The authors declare no conflict of interest.
Publisher’s Note: IMR Press stays neutral with regard to jurisdictional claims in published maps and institutional affiliations.