- Academic Editor
Cancer whose major problems are metastasis, treatment resistance, and recurrence is the leading cause of death worldwide. Tumor-initiating stem cells (TiSCs) are a subset of the tumor population responsible for tumor resistance and relapse. Understanding the characteristics and shared features between tumor-initiating stem cells (TiSCs) and long-lived postmitotic cells may hold a key to better understanding the biology of cancer. Postmitotic cells have exited the cell cycle and are transitioned into a non-dividing and terminally differentiated state with a specialized function within a tissue. Conversely, a cancer cell with TiSC feature can divide and produce a variety of progenies, and is responsible for disease progression, tumor resistance to therapy and immune system and disease relapse. Surprisingly, our comprehensive evaluation of TiSCs suggests common features with long-lived post-mitotic cells. They are similar in structure (primary cilia, high mitochondrial content, and being protected by a barrier), metabolism (autophagy and senescence), and function (immunoescape and/or immune-privileged by a blood barrier). In-depth exploration showed how mitochondrial metabolism contributes to these shared features, including high energy demands arising from ciliary and microtubular functionality, increased metabolic activity, and movement. These findings can assist in decoding the remaining properties which offer insights into the biology of TiSCs, with potential implications for enhancing cancer treatment strategies and patient prognosis.
There is still a long way to definite cancer treatment. One of the greatest hurdles to this end is tumor-initiating stem cells (TiSCs) (also known as cancer stem cells) [1]. They are a component of the tumor microenvironment (TME), enhancing the treatment resistance [2] and immunoescape of cancer cells [3]. In addition, TiSCs can modulate the TME to support cancer progression and promote immunosurveillance [4]. Compared to normal stem cells, TiSCs have distinct phenotypes and capabilities, as follows: TiSCs and normal stem cells are distinct populations of cells with differing characteristics and roles. While they share some similarities, they also exhibit critical differences, especially in terms of their behavior, regulation, and implications for health and disease. Normal stem cells found in various tissues and organs, but TiSCs are a subpopulation of cells within a tumor which are arise from genetic mutations or alterations in normal stem cells or progenitor cells, causing them to acquire properties of uncontrolled growth [5]. Normal stem cells contribute to the replacement of damaged or dying cells and help maintain tissue homeostasis [6]. TiSCs are thought to be responsible for tumor initiation, growth, and recurrence via possessing stem cell-like properties and contribute to the heterogeneity of cancer cells within a tumor [7]. Normal stem cells have self-renewal capacity including dividing and producing both identical stem cells and differentiated cells; Similarly, TiSCs can also self-renew and give rise to more TiSCs and differentiated cancer cells. Normal stem cell divisions are tightly regulated via intrinsic mechanisms to prevent excessive proliferation and maintain tissue integrity via following specific cues from the microenvironment (stem cell niche). However, TiSCs undergo uncontrolled proliferation, leading to tumor formation and growth [8]. Normal stem cells typically maintain genetic stability and exhibit low rates of genetic mutations [9], whereas TiSCs contribute to tumor heterogeneity [10]. The origin of TiSCs and how they are regulated are still not clear. To decode the characteristics of TiSC, a potential approach is to detect its counterparts in nature.
Postmitotic cells (PoMiCs) refer to cells that do not exhibit mitosis
and cell division after the completion of fetal development. In fact, they are
mature cells no longer capable of undergoing mitosis compared to
intermitotic cells [11]. Examples of long-lived PoMiCs are central
nervous system (CNS) neurons [12], skeletal muscle cells [13], retinal pigment
epithelium (RPE) [14], renal podocytes [15], and pancreatic islet cells [16].
Most mammalian PoMiCs (such as neurons) may accumulate high amounts of DNA damage
during their lifetime [17]. In fact, the presence of dedicated repair mechanisms
in the cycling neuroprogenitor cells would also prevent these lesions from
accumulating during neurodevelopment. However, the enhanced DNA repair mechanisms
are absent in postmitotic neurons, leading to cellular dysfunction through time
[18]. The hallmark model for neurodegeneration results from DNA damage
accumulation in the absence of DNA repair genes which results in neuronal
dysfunction and, eventually, cellular death [19]. Recent studies have
demonstrated that PoMiCs can obtain several key characteristics of senescent
cells. For instance, it is shown that the neurons have several
senescence-associated properties, including the high activity of
In the context of tumor development, PoMiCs and other normal cells play distinct roles that contribute to the complexity of tumor growth and progression. PoMiCs do not directly contribute to tumor growth through cell division. However, they can be affected by the tumor microenvironment and may undergo changes in response to signals from neighboring tumor cells. For example, surrounding PoMiCs may experience altered gene expression patterns or changes in their microenvironment due to factors released by tumor cells. Normal cells within the tumor microenvironment include various cell types, including immune cells, fibroblasts, and endothelial cells among others [22]. These cells interact with tumor cells and contribute to tumor development in several ways [23]. Conversely, vulnerabilities revealed by tumor cells when in a plastic or stem-like state may be therapeutically exploited, i.e., by transforming them into PoMiCs [24].
A special characteristic linking TiSCs to PoMiCs is primary cilia. Primary cilia are hair-like, membrane-bound organelles responsible for a variety of functions in human cells, such as fluid flow (by motile cilia on ependymal cells, oviduct cells, and renal tubular cells), cell division (by nonmotile cilia on renal tubular cells), photoreception (by nonmotile cilia on retinal rod and cone cells), and olfaction (by nonmotile cilia on olfactory epithelium) [25]. Although PoMiCs have mostly cilium, the role of primary cilia in well-differentiated and non-dividing postmitotic cells is not clarified. This article highlights how primary cilia can be involved in the immune privilege of PoMiCs and TiSCs. Disruption of primary cilia function or distortion of its structure can potentially lead to autoimmune diseases and cancer development.
This study was conducted to determine why most of the PoMiCs are accommodated in an immunosuppressive barrier environment. Briefly, the highly sophisticated cells, PoMiCs and TiSCs, have certain commonalities. The molecular mechanism, micro-anatomic structure, behavior, and function such as being immune-privileged, would be one area of similarity. The cilia and mitochondrial enrichment of these cells are two additional characteristics that these two cells share and which we mentioned as the article’s major topic.
Considering the above-mentioned similar phenomena, structures, and behaviors, is there any lesson from long-lived PoMiCs to target TiSCs for efficient cancer immunotherapy? Are there any common antigenic proteins between these two immune-privileged cell types (TiSCs and PoMiCs)? Are there any genetic, histologic, structural, or even physiologic similarities (such as mitochondrial number, degree of hypoxia, demand to energy, polarity, or status of microtubular structure) as a clue for shaping TME into a landscape that favors TiSCs for immune system accessibility, or to activate apoptosis in TiSCs? The answer to these questions can help to overcome the TiSCs, as the main actors of cancer resistance, progression, and recurrence.
In the human species, motile and nonmotile cilia are found on almost all cells in different tissues and organs; however, ciliary enrichment is a characteristic of specific tissues. Examples of ciliary-rich cells include CNS neurons, mature muscle cells, nephrons, photoreceptors in retina, and mature spermatocytes. Primary cilia are enriched both in non-dividing cells that can re-enter the cell cycle and in terminally differentiated cells without this ability. Table 1 represents the ciliary-rich cells and tissues and the corresponding syndromic ciliopathies.
Tissue | Function | Disease |
Brain (neuron) | Sensation, move, feelings | Joubert Syndrome |
Testis (sperm) | Male fertility | Male infertility |
Retina (photoreceptors) | Vision | Retinitis pigmentosa, LCA, cone/rod atrophy, retinal Degeneration |
Kidney (podocytes and nephron epithelium) | Urinary excretion | ADPKD, Nephronophthisis (NPHP), BBS, MKS, Alstrom syndrome, renal Cyst, renal cell carcinoma |
Ovarian fallopian tubes | Ovum movement from ovary to uterus | Female Infertility |
Melanocyte | Neural crest late migratory pathway | Benign hyperproliferation and abundant nevi in BBS patients predispose to skin cancer |
Olfactory bulb | Frontal lobe structure and nasal secretary | Single central incisor or agenesis of corpus callosum, hydrocephaly |
Olfactory epithelium | Smell | Anosmia |
Pancreas | Islet cells development | Hypoplastic pancreas, obesity, diabetes mellitus |
Ear | Stereocilia and kinocilia | Deafness (sensorineural hearing loss), stereocilia bundles (hair cell) disorientation |
Abbreviations: ADPKD, autosomal-dominant polycystic kidney disease; BBS, Bardet-Biedl syndrome; LCA, Leber congenital amaurosis; MKS, Meckel-Gruber syndrome, Medullary sponge kidney; NPHP, Nephronophthisis.
Since primary cilia in PoMiCs are not necessary to prevent cell cycle re-entry, the main question is whether cilia have any specific function in these cells. Except for comprising the survival of cells under stress, primary cilia have no proven critical function outside the embryonic process [26]. Primary cilia appear to be necessary for cells that re-enter the cell cycle to replace necrotic or apoptotic cells (such as after ischemia or toxic insults) to stop proliferative responses and resume tissue-specific tasks [27] (Fig. 1). An example of this particular function is human embryonic stem cells that produce primary cilia in order to develop into neuroectoderm and avoid recycling [28]. On the other hand, it has been demonstrated that renal tubular epithelial cells without primary cilia cannot provide an appropriate response to ischemic injury and produce cysts upon the insult [29].
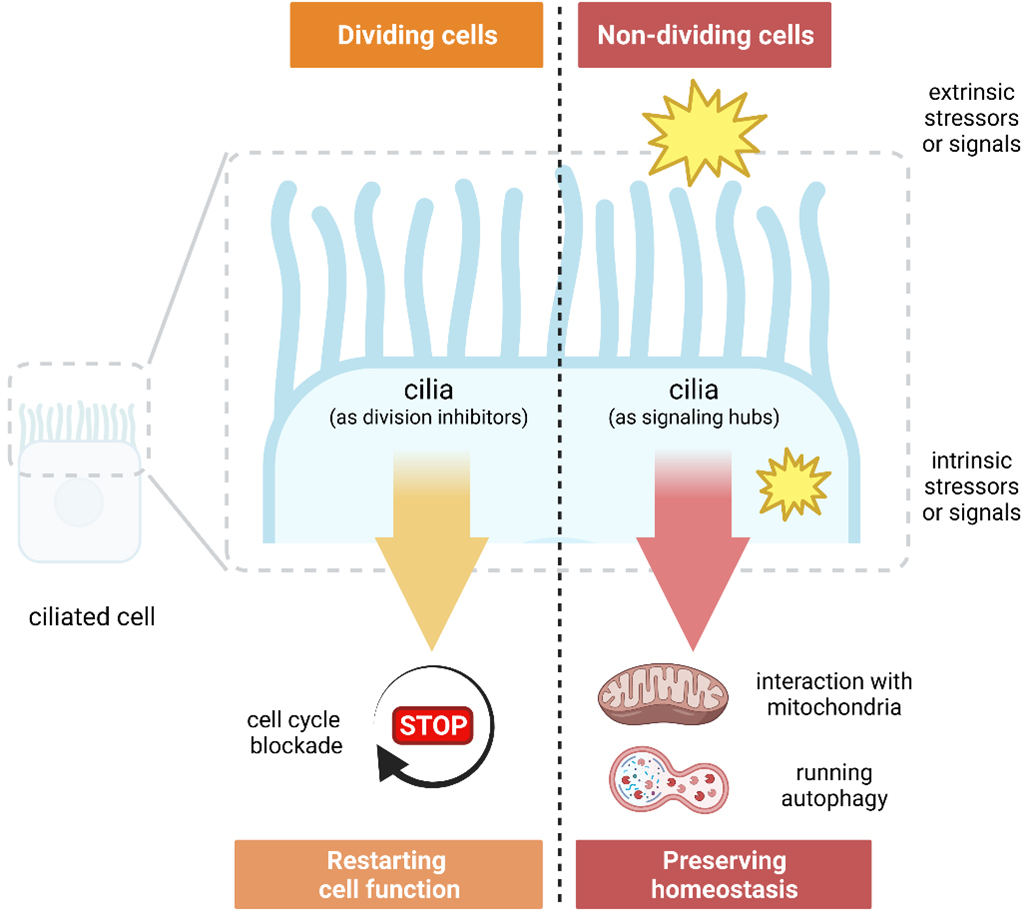
Distinct roles of cilia in dividing and nondividing cells.
The role of primary cilia is less evident in cells that cannot proliferate in response to injury to replace damaged tissue. Primary cilia may sense and conduct repair reactions to ensure cell survival by preventing tissue degeneration brought on by gradual cell loss or induced senescence [30]. Primary cilia’s detailed participation in essential chemical processes that sustain cellular homeostasis lends credence to the hypothesis that they function as a signaling hub to react to shocks that threaten cellular integrity. Recent evidence has linked DNA damage responses, autophagy, and mitochondria to centrosome-cilium complex, microtubular proteins, and the molecules linked with cilia [31]. Theoretically, in nondividing cells where replication-induced DNA damage is less important, a signaling hub external to the nucleus that integrates nutritional status, energy supply, and exposure to harmful events may coordinate appropriate responses. In order to prevent the activation of antagonistic cellular programs and responses, mTOR and Hippo signaling cascades, which are regulated by cilia, are coordinated [31]. It has been demonstrated that primary cilia in PoMiCs can interact with mitochondria and are involved in mechanistic pathways regulating autophagy [31]. In brief, primary cilia in postmitotic nondividing cells are well-organized to form a signaling hub outside of the nucleus to preserve cellular homeostasis in response to different insults, including DNA damage (Fig. 1).
Based on recent findings, cilia might hypothetically be responsible for several functions of non-dividing and PoMiCs. Those functions include sensing nutritional status, energy supply, cell shape, cell volume, and cell polarity. In addition, as noted above, cilia are involved in response to damaging events that endanger cell survival and prevent senescence and cell death. If cilia play such a role, they must coordinate responses following (nuclear or mitochondrial) DNA damage, such as that brought on by the release of reactive oxygen species (ROS) during hypoxia, the activation of autophagy during nutritional deprivation, or the induction of apoptosis in the case of irreversible damages. A central hub, as opposed to several disorganized, and sometimes hostile subsidiaries, is unquestionably required when deciding whether to live or perish. Since replication stress is not a concern in cells that are not dividing, it may make sense to locate such a center outside the nucleus. Emerging evidence points to cilia’s potential to be crucial in a cellular emergency [31]. However, under no cellular stress, the purpose of cilia in non-dividing cells might not be revealed. Non-dividing cells’ cilia may also play a role in subtler phenotypes, such as the flow-dependent control of potassium secretion in distal nephron epithelial cells [32].
It has been established that numerous signaling elements are evolved as negative regulators of ciliogenesis during the cell cycle, while other molecules and proteins have been developed to stabilize cilia in non-dividing cells [33]. Considering the pivotal and essential presence of cilium in PoMiCs, and the significant overlap and correlation between the organs and tissues containing long-lived PoMiCs and the ciliary-rich ones, we may name this cluster group as long-lived postmitotic ciliary-rich (LPC) cells.
TiSCs per se are immune privileged and highly treatment-resistant, increasing the chance of tumor recurrence [34]. These features of TiSCs are similar to LPC cells. Emerging evidence addresses the importance of primary cilia in TiSCs biology (Table 2, Ref. [35, 36, 37, 38, 39, 40, 41]). Primary cilia as non-motile, antenna-like structures play important roles in cell signaling, sensory perception, and cellular communication; are involved in various signaling pathways which are associated with growth, development, and homeostasis. There are some interesting points to address a direct role for cilia in promoting cellular senescence and senescence-associated secretory phenotype (SASP) in cancer cells, including:
- Cilia-Mediated Signaling - Primary cilia play roles in the Hedgehog signaling pathway which some of its components are implicated in cellular senescence and aging processes. Alterations in cilia-mediated signaling could potentially affect cellular senescence [42].
- Cell Cycle Regulation - Primary cilia are involved in regulating the cell cycle and proliferation. Dysregulation of these processes can contribute to cancer and cellular senescence. However, the exact mechanisms linking primary cilia to the induction or promotion of cellular senescence are not well-established [43].
- Mechanosensory Functions - Primary cilia can function as mechanosensors, responding to changes in the mechanical environment around cancer cells. Mechanical stress and changes in the stiffness of cancer tissue have been implicated in cellular senescence. There could be indirect effects of cilia-mediated mechanosensation on senescence pathways [44].
Year | Study | Findings |
2009 | Han et al. [38] | The cerebellar granule neuron precursors develop into medulloblastoma via cilia-Shh axis |
2015 | Gate et al. [35] | CD15 |
CD15 | ||
2017 | Guen et al. [39] | Primary cilia ablation is sufficient to repress shh signaling, the stemness of MaSCs, and the tumor-forming potential of MaTiSCs |
2020 | Jeng et al. [40] | The self-renewal of CSCs is regulated by the Shh/Smoothened receptor (SMO)/Glioma-associated oncogene homolog I (GLI) signaling pathway |
2021 | Wilson et al. [37] | Cilia activate the stemness of mammary TiSCs by inhibiting GLIS2 |
2021 | Goranci-Buzhala et al. [36] | Cilia suppression induces glioma stem cells renewal |
Cilia rescue suppresses glioma stem cells renewal and promotes differentiation | ||
2021 | Chippalkatti et al. [41] | Oncogenic K-Ras contributes to cancer cell stemness via disrupting fundamental polarized signaling and asymmetric apportioning processes |
Abbreviations: CSC, cancer stem cells; GLIS2, GLIS family zinc finger 2; MaSCs, Mammary stem cells; Shh, Sonic Hedgehog; (Ma)TiSCs, (mammary) tumor-initiating stem cells.
A robust example linking cilia to TiSCs can be found in medulloblastoma. It is
believed that medulloblastoma originates from neural stem cells upon aberrant
activation of the Sonic hedgehog (Shh) signaling pathway [45]. The upstream
mediators of the Shh pathway are Smo, ptch, and Gli, mainly located on the
primary cilia [46]. Therefore, Shh signaling in medulloblastoma depends on the
functional primary cilia. Gate et al. [35] found a distinct subset of
medulloblastoma cells that were CD15
The relationship between primary cilia and cancer is complex and an active area of research [47]. The presence or absence of cilia can vary widely depending on the type of cancer, the stage of cancer (cancer heterogeneity), and the specific genetic and molecular characteristics of the cells involved [47]. Some mammalian cells are enriched by primary cilia which are sensory organelles extending from their surface. In some cases, cancer cells can lose their primary cilia due to the disruptions in the molecular pathways that govern cilia formation and maintenance [47]. This loss of primary cilia has been observed in certain cancer types. In this regard, there are some general observations:
- Cilia Loss in Some Cancers: In certain cancer types, including “aggressive or highly proliferative cancers”, the loss of primary cilia has been reported. This loss is associated with alterations in signaling pathways (e.g., Hedgehog pathway), that are important for cilia formation and maintenance [48].
- Cilia Retention in Other Cancers: Cancer cells with slower growth rates or those that arise from cilia-enriched tissues where primary cilia play important roles in (normal) cell function may retain primary cilia [49].
- Therapeutic Implications: The presence or absence of primary cilia in cancer cells could have therapeutic implications, as some targeted therapies have been developed to exploit the cilia-dependent signaling pathways in certain cancer types [49].
For instance, Kras may be directly suppressing the development of primary cilia [50] Similar to this, spontaneous clear renal cell carcinoma, which is characterized by mutations in the tumor suppressor von Hippel-Lindau(VHL), a protein hypothesized to be involved in cilia maintenance, similarly exhibits a markedly decreased percentage of ciliated cells [51]. These instances may thus serve to support the presumably intuitive theory that cilia inhibit and are incompatible with cell growth. However, it has been demonstrated that cilia are necessary for the expansion and maintenance of specific cell populations as well as the development of specific cancer types, including those that depend on Shh signaling [44].
It is critical to stress that the function of primary cilia in cancer is probably context/genetically specific, depending on the molecular and microenvironmental properties of the cancer cells. However, this distinction appears to be founded on and related to the cilium enrichment of the normal cell where carcinogenesis took place. Therefore, primary cilium is not only important in carcinogenesis, but also it may be considered as a marker for the cancer treatment strategy. This important note may interpret the cilia behavior in medulloblastoma or glioblastoma, and what is observed in breast cancer [44, 52, 53].
Epithelial-to-mesenchymal transition (EMT) is a critical molecular program that
regulates several aspects of tumor development. It is an essential developmental
process that enables immotile epithelial cells to acquire mesenchymal features
such as migration, transformation of the microenvironment, and invasion [54, 55].
Reactivation of EMT has also been shown in adult tissue regeneration and
diseases, including cancer [55, 56, 57, 58, 59]. There is extensive evidence identifying EMT
as a driver of cancer progression and metastasis from human patients and mouse
models [60, 61, 62, 63, 64, 65, 66, 67, 68, 69, 70]. One functional aspect of EMT induction is the regulation of
stemness [71]. Albeit, different EMT regulators have different outcomes in terms
of induction or repression of stemness depending on the context [55, 67, 71, 72, 73, 74, 75, 76, 77].
EMT also affects the TME from a cilia standpoint; for instance, EMT transcription
factors can induce ciliogenesis via FGF signaling, promoting stemness and
proliferative capacities during mammary gland development as well as in breast
cancer [37]. EMT factors downstream of TGF-
Like the PoMiCs and TiSCs, certain blood barriers can contribute to creating immune-privileged or immunosuppressed environments in specific tissues [80]. The concept of immune privilege refers to sites in the body where immune responses are intentionally downregulated or suppressed to protect delicate structures or maintain specific functions. Blood barriers, like the blood-brain barrier (BBB) and the blood-retinal barrier (BRB), play roles in establishing immune privilege in their respective tissues. For instance, the BBB is a specialized barrier formed by endothelial cells lining blood vessels in the brain, and limits the passage of many substances, including immune cells and molecules, from the bloodstream into the brain [81]. The immune privilege of the CNS not only helps prevent widespread inflammation and immune-mediated damage within the brain, but also poses challenges in treating certain brain infections or immune-related disorders. This challenge mimics the behavior of the highly specific TiSCs which are protected from immune cells as well as chemo-radiotherapies [82]. Like the BBB, the BRB helps protect the sensitive structures of the eye from immune-related damage; this immune privilege is particularly important for maintaining vision and retinal function [83]. Overall, the selective permeability of the blood barriers contributes to reduced immune activity which helps prevent unnecessary inflammation and immune responses that could disrupt the normal functions of these tissues. Immune privilege is not absolute, and immune responses can still occur within these tissues under certain circumstances. Additionally, while blood barriers contribute to immune privilege in certain contexts, immune privilege can also be influenced by other factors, such as local immune regulation and tissue-specific mechanisms [84].
Ever since, various epithelial and endothelial blood-tissue barriers have been detected, including the blood-brain barrier (BBB), the blood-cerebrospinal fluid barrier (BCSFB), the blood-retina barrier (BRB), the blood-testis barrier (BTB), the glomerular filtration barrier, peri-islet basement membrane (and subjacent interstitial matrix in the pancreas), the blood-placenta barrier (BPB), etc. These highly specialized structures provide size and charge barriers to prevent high molecular weight proteins in the plasma (e.g., albumin and globulins, including gamma-globulins and immunoglobulins) from passing through the membrane and being delivered to the target tissues. For example, BBB is made up of capillary endothelial cells and basement membrane, neuroglial membrane, and glial podocytes and functions in synchronicity to limit the entry of various substances into the cerebral blood flow, subsequently the brain parenchyma.
These highly selective barriers between blood and different tissues are required to maintain tissue homeostasis. Blood barriers are mainly composed of capillary endothelial cells and basement membrane (basal vascular component), glial podocytes (ciliary and microtubule-based structure), and a local organ membrane (neurological, tubular, seminiferous epithelium, retinal pigment epithelial, etc.). Although the precise mechanisms are still not completely understood, the protruding primary cilia—from the surface of endothelial cells—seem to play a crucial role in the regulation of blood barriers [85]. Therefore, it appears that there is a consistency between the anatomical position of LPC cells and the presence of blood barriers in humans and, generally, in mammals. This is an interesting phenomenon from developmental biology and evolutionary points of view.
The function of blood barriers may define and determine why these highly functional, specific, and differentiated cells (i.e., LPC cells) should be separated and protected from blood exposure. For instance, avoidance from the immune system, in particular humoral immunity, is a well-known function of blood barriers; Therefore, the blood barrier can provide those tissues with immune privileges to keep away from autoimmunity. Knowledge of physiological mechanisms contributes to comprehending the underlying pathophysiological mechanisms. One main function of the blood barrier is limiting the entry of immune cells and mediators into the target tissues. It means that blood barriers function to protect the aforementioned organs from immune system access. Therefore, the LPC cells (in CNS, retina, testes, pancreatic islets, and renal glomeruli) are likely immune-privileged. Even foreign antigens accessing these tissues may not generally trigger immune responses [86]. Hence, the LPC cells are accommodated in an “immunosuppressive barrier environment”. This spectacular environment of immunosuppressive barrier is anatomically structured and evolutionary conserved in the human species and most mammals. As such, primary impairment of blood barriers is a possible mechanism of autoimmune diseases.
Blood barrier plays a key role in protecting from humeral immunity and maintaining tissue homeostasis, whereas cilia are involved in its generation, integration, and regulation through different signaling pathways. However, several aspects and main questions about the mechanism still need to be understood. Nonfunctional cilium-related proteins may damage the blood barrier via HH, Notch, Wnt, and other signaling pathways [4]. It is proposed that ciliopathy is molecularly caused via damage to intercellular junctions or paracellular transport, including tight junctions and adherens junctions [87, 88]. Blood barrier dysfunction is well-documented in some ciliary diseases, while the knowledge of ciliopathies is expanding. Blood barriers comprise several transmembrane proteins, including connexins [89]. A dysfunction in any of the proteins which play a role in ciliary signaling may lead to a disintegration of the blood barrier. The mechanisms of how cilia function in maintaining the integration of blood barriers help to treat blood barrier-related diseases, although it is currently elusive [85].
In brief, the microtubule-based primary cilia, as a component of PoMiCs, are both structurally and functionally crucial components of the blood barriers. Ciliary signaling pathways are likely involved in regulating this anatomic barrier. In addition, as a part of the vascular barrier, endothelial cilia cross-talk via functioning in mechanisms including cell migration, blood flow sensing, and nitric oxide (NO) and calcium signaling, which are also some known molecular mechanisms in carcinogenesis and tumor biology [90, 91].
TME consists of tumor-associated macrophages (TAMs), myeloid-derived suppressor
cells (MDSCs), regulatory T-cells (Tregs), stromal cells, cancer-associated
fibroblasts (CAFs), and endothelial cells [92, 93]. These cells support cancer
progression, treatment resistance, and immunoescape [94, 95]. TiSCs have a
pivotal role in tumor initiation and progression and are also critical in immune
escape and crucial for treatment resistance in response to chemotherapy and
radiotherapy [96, 97]. The main concern would be whether and how the TME assists
TiSCs’ survival from therapies provocations and supports upcoming cancer cell
progression. In answering these important questions, TiSCs intriguingly instruct
and shape TME into a setting that provides TiSCs with immunosuppression and
propagation [4]. TiSCs may not be recognized and eliminated by the immune system
as they not only change the composition but also alter the functional properties
of immune cell subsets. This big alteration in the immune cell subsets is
implemented by secreting soluble molecules (e.g., Periostin, TGF-
In addition to hypoxia, a few other significant microenvironmental cues, such as ECM, TiSCs/stroma cells, and immune cells, have an influence on the EMT program and have been shown to affect tumor formation, progression, and therapeutic response in human malignancies [115]. Recruiting evidence addresses that cilia, as sophisticated gestures, are present in the micro-anatomical level of TME and TiSCs (see section 3). Section 3 outlines how cilia are crucial to protect the PoMiCs from a self-immune system. As such, cilia in TME and TiSCs might contribute to the immune privilege of tumors. Given that both mitotic spindles and cilia are composed of microtubules (with the same molecular apparatus and organization), the close connection between cilia and the cell cycle progression is not unexpected. Given that mitotic spindle is necessary for cell cycle progression and cytokinesis, cilia block cells from entering the cell cycle. Cilia would be utterly unnecessary, for example, on terminally differentiated cells that cannot re-enter the cell cycle if this was their only function. Ciliogenesis in cancer cells is dependent on the cancer type. Unsurprisingly, most cancer cells lack cilia because they serve as gatekeepers to stop cell cycle re-entry [116, 117, 118]. Nevertheless, several others exhibit increased numbers of primary cilia [119]. Ciliation might be varied even in a single cancer type. For instance, Emoto et al. [120] detected primary cilia on one-fourth of pancreatic ductal adenocarcinoma cases. They found that tumors with cilia overexpression were more prone to lymph node metastasis and had poorer overall prognosis. It has been demonstrated that the tumor suppressor/oncogene fingerprint of cancer cells regulates ciliogenesis. For example, the Shh, LKB1, Wnt, and Hippo pathways are just a few of the significant tumor-promoting pathways that regulate ciliogenesis [47]. Also, the loss of primary cilia results in apoptogenic stimuli, which are responsible for mitochondrial-dependent apoptotic cell death in differentiated thyroid cancers [49].
Cilia are dependent on proper mitochondrial metabolism to maintain their structure and function. It has been demonstrated that mitochondrial dysfunction and ROS accumulation can decrease cilia number and/or length in the inner medullary collecting duct cells. In addition, mitochondrial dysfunction can lead to ciliary dysfunction by reducing cellular adenosine triphosphate (ATP) content [121]. In support, Ignatenko et al. [122] found similar findings in astrocytes. They demonstrated that depletion of mitochondrial DNA can modify cellular cilia from the primary type into motile type. This change can impair the BBB integrity, which depends on primary cilia to work correctly [123]. Collectively, mitochondrial dysfunction can lead to blood barrier dysfunction. This notion was demonstrated by Doll et al. [124] in a mouse model with a cerebrovascular accident. As alluded in section 3, a defective blood barrier can expose the already protected antigens to the bloodstream, which aberrantly activates the immune system against autoantigens that results in autoimmune diseases. Therefore, mitochondrial impairment and the resultant ciliary dysfunction might contribute to the development of autoimmune diseases. Impaired mitochondrial dysfunction has been demonstrated in patients with multiple sclerosis [125], even in their peripheral blood mononuclear cells [126]. The next two sections illustrate the similarities between LPC cells and TISCs.
The crucial role of mitochondria in apoptotic pathways [127] and cancer cell survival [94, 128] have been known for ages. Cilia and mitochondria are the two cell organelles that directly interact with each other. As noted in Section 4, appropriate mitochondrial metabolism is essential for cilia to properly maintain their structure and function. As such, PoMiCs homeostasis relies on mitochondrial metabolism and dynamics. Iwata et al. [129] demonstrated that mitochondrial dynamics can determine the fates of daughter cells resulting from neural stem cell division. They realized that increased mitochondrial fission promoted the daughter cells’ conversion to neurons, whereas mitochondrial fusion led to preserving stemness [129]. The dependence of PoMiCs on mitochondrial metabolism is in common with TiSCs. It has been demonstrated that TiSCs have high mitochondrial content to support their survival [130], function [131], and treatment resistance [132, 133]. The cilia-mitochondria interaction is bidirectional. The cilia-associated molecules involved in microtubule dynamics also affect mitochondrial trafficking [31]. Mitochondria are not essential for ciliogenesis [134], but the function and energy source of primary cilia may be at least partly dependent on mitochondrial ATP. It has been shown that ATP consumption depends on the beating frequency [135] and the ATP production by glycolysis occurs in the ciliary axoneme [136]. According to structural studies of photoreceptors, mitochondria can be found adjacent to the connecting cilium and basal body, indicating that ATP diffusion into the outer segment of rods serves as a source of energy [137]. Since motor proteins that consume ATP drive intraflagellar transport, ciliary processes may be affected by ATP deprivation brought on by, for instance, hypoxia or a lack of glucose.
Another common feature of PoMiCs and TiSCs is the enhanced autophagy process. Autophagy is vital for PoMiCs that are unable to divide to maintain their homeostasis [138], and for TiSCs to survive in the state of the harsh TME and different treatment [139]. Mitochondria can regulate autophagy in normal [140] and cancer cells [94]. Senescence is another common characteristic of PoMiCs and TiSCs. It is a mechanism by which TiSCs stop their cell cycle to provide efficient resistance against radiotherapy and anticancer agents [141]. It has been demonstrated that senescence in cancer cells is conducted by mitochondria. Hubackova et al. [142] demonstrated that targeting cancer cells’ mitochondria by mitochondria-targeted tamoxifen can effectively reverse the senescence by suppressing the level of adenine nucleotide translocase-2. Although senescence in PoMiCs is associated with progressive mitochondrial dysfunction [143], recent evidence has identified that a proper interaction between mitochondria and endoplasmic reticulum is essential to conduct senescence [144]. The common point of TiSCs with PoMiCs in reliance on mitochondrial metabolism can guide in identifying the uncharted mechanisms that TiSCs apply to improve their mitochondrial content.
This section provides the common mediator of primary cilia and mitochondrial metabolism to better understand their interaction. Voltage-dependent anion channel 2 (VDAC2) is localized at centriolar satellites to promote ciliary maturation, but VDAC1 and VDAC3 have a detrimental effect on ciliogenesis; in addition to their known roles in mitochondrial bioenergetics during ciliogenesis, all three VDAC isoforms have been found in a non-mitochondrial centrosomal pool, indicating a function [145]. Mammalian sperm illustrates how cilia can attract and assemble mitochondria. ODF2 (outer dense fiber protein 2) attracts mitostatin/trichoplein (two proteins preventing cilia formation during the cell cycle), promotes cilia assembly during quiescence, and controls mitochondrial-endoplasmic reticulum binding via Mitofusin-2 (MFN2) [31]. Numerous benign and metastatic cancers, including renal cell cancer (RCC), can develop due to mutations in the VHL tumor suppressor. By maintaining microtubule stability, VHL, which seeks to degrade HIF1, encourages ciliogenesis [146, 147]. The loss of cilia has not, however, always been linked to the deletion of VHL, and VHL knockout in mice does not result in RCC. In fact, the combined deletion of three genes, namely VHL, Trp53, and Kif3a, is necessary to develop cystic and neoplastic lesions [148, 149]. Like VHL tumor suppressor, TSGA10 (testis-specific gene 10 protein) is a sperm (testis)-specific protein with crosstalk between postmitotic ciliary-rich cells/tissues and progressive stages of cancers via mitochondria. As a cancer-testis antigen, TSGA10 is a ciliary-centrosomal protein, which is also overexpressed in cancers (solid tumors and leukemias), interacts with HIF1-alpha and ODF2, and is involved in the correct arrangement of the mitochondrial sheath in spermatozoa [150, 151, 152, 153, 154].
Therefore, cilia-associated mitochondrial proteins may direct calcium release, mitochondrial depolarization, and mitophagy induction in response to cellular stress or may recruit mitochondria to the area of basal bodies or connect cilium to increase ATP delivery to the ciliary axoneme. However, if they are close, cilia would be vulnerable to ROS produced by mitochondria in reaction to oxygen deprivation. Recent studies relate DNA damage responses, autophagy, and mitochondria to molecules linked with cilia [31]. Therefore, in parallel with cilia, mitochondria might also be the crosstalk between non-dividing long-lived postmitotic cells and therapy-resistant tumor-initiating stem cells.
This review demonstrated that TiSCs are similar to PoMiCs in terms of structure (primary cilia and high mitochondrial content), metabolism (autophagy and senescence), and function (immunoescape). This finding can assist in decoding the remaining features of TiSCs and improve the treatment response and overall prognosis of patients with cancer. Besides, this review illustrated how primary cilia might improve the immune privilege of TME and TiSCs. Given the role of cilia-associated signaling in treatment resistance, targeted therapies disrupting the corresponding signaling pathways can improve the treatment response of cancer cells. As noted, active mitochondrial metabolism is required to maintain the function and structure of cilia in cancer cells. Therefore, developing targeted anti-mitochondrial therapies can help to reduce cells resistance. Future research must concentrate on the role of cilia in maintaining cellular homeostasis even though ciliogenesis and ciliary disassembly have been thoroughly researched and the identification of a large number of molecules. Upcoming studies are interesting to get around the constraints provided by the growing cancer cells commonly employed to characterize the function of primary cilia via in vivo techniques or primary cell cultures.
BB performed literature search, drafted the primary manuscript, and supervised the whole study as the expert; FTH revised the manuscript, confirmed the final manuscript, and prepared the figures; HF has contributed in acquiring and interpreting data from the reviewed literature and wrote a part of the manuscript; PF contributed to the manuscript via providing an interpretation of data in the second read; HF and PF reviewed and revised the manuscript; MG performed literature research and prepared the tables. All authors have read and approved the final manuscript, contributed to editorial changes in the manuscript, participated sufficiently in the work to take public responsibility for appropriate portions of the content and agreed to be accountable for all aspects of the work in ensuring that questions related to its accuracy or integrity.
Not applicable.
Not applicable.
This research received no external funding.
The authors declare no conflict of interest.
Publisher’s Note: IMR Press stays neutral with regard to jurisdictional claims in published maps and institutional affiliations.