- Academic Editor
Background: The production of reactive oxygen species (ROS) in animals and cells often results from exposure to low-intensity factors, including magnetic fields. Much of the discussion about the initiation of oxidative stress and the role of ROS and radicals in the effects of magnetic fields has centered on radical-induced DNA damage. Methods: The DNA concentration in the final solution was determined spectrophotometrically. Typing of the polymorphic variant rs1052133 of the 8-oxoguanin DNA glycosylase (hOGG1) gene was performed by polymerase chain reaction. An enzyme immunoassay was performed to determine the level of 8-oxyguanine in DNA. To process samples exposed to an alternating magnetic field, the authors developed a device for the automated study of biological fluids in an alternating magnetic field. The content of hydrogen peroxide in aqueous solutions of DNA was determined using the spectrophotometric method. Results: It was experimentally determined that an increase in the concentration of hydrogen peroxide in an aqueous medium by 3–5 times under the action of a low-frequency magnetic field reduces the resistance of the genomic material to oxidative modification and the accumulation of 8-oxyguanine in DNA. A model is proposed for the mechanism of action of a low-frequency magnetic field on aqueous solutions of nucleic acids and proteins, which satisfies the model of a chemical oscillator for the transformations of reactive oxygen species in an aqueous medium. The model illustrates the oscillating nature of the processes occurring in an aqueous solution of DNA and makes it possible to predict changes in the concentration of hydrogen peroxide in an aqueous solution of biopolymers, depending on the frequency of the acting low-intensity magnetic field. Conclusions: The key element in the mechanisms involved in the effects of low-intensity magnetic field on living systems is the occurrence of ROS generation in the aquatic environment of chemical oscillators, in which the competition of physical and chemical processes (electron transfers, reactions of decay and addition of radicals, spin magnetically induced conversion, synthesis, and decay of the longest-lived form—hydrogen peroxide) is controlled by a magnetic field.
The most common product of oxidative DNA damage under oxidative stress is
8-oxo-7,8-dihydroguanine (8-oxoG), which is the main biomarker of genome
conformational rearrangements [1]. According to ESCODD (European Standards
Committee on Oxidative DNA Damage), the level of endogenous 8-oxoG in DNA is
about one 8-oxoG per 10
During excisional repair, damaged areas are cut out of the DNA strand and the
gaps are filled with intact material. In humans, 11 glycosylases have been
isolated and described. These are 8-oxoguanine-DNA-glycosylases (hOGG1, EC
3.2.2.23), which remove 8-OH-guanine, leaving behind a single-strand break [3, 4]. As a result of the 8-oxoguanine-DNA-N-glycosylase (hOGG1, EC 3.2.2.23)
enzymatic function, the sequential hydrolysis of the N-glycosidic bond occurs
from the 3
The production of reactive oxygen species (ROS) in animals and cells often results from exposure to low-intensity factors, including a magnetic field [6, 7, 8, 9, 10, 11, 12, 13, 14, 15]. ROS cause a shift in the state of the pro-/antioxidant system, thereby contributing to the formation of varying degrees of oxidative stress, which is one of the reasons for the disruption in the hereditary material structure [16, 17]. The corresponding hypotheses and experimental data are summarized and discussed in several reviews on this topic [6, 18, 19, 20, 21]. Much of the discussion about the initiation of oxidative stress and the role of ROS and radicals resulting from the effects of magnetic fields is focused on DNA damage caused by radicals [20, 22, 23].
Biological systems are constantly under the influence of natural and artificial sources of magnetic fields (MFs). In the course of evolution, they have developed mechanisms for perceiving information about the state of the environment through interactions with the Earth’s magnetic field. Indeed, biological systems are highly sensitive to changes in the magnetic background as an evolutionarily justified factor that contributes toward their survival. At present, there are a sufficient number of experimental studies on the impact of low-frequency MFs on biological systems; nevertheless, the nature of these phenomena is not completely clear [18, 24, 25, 26].
The impact of MFs on living systems, especially those in the low-frequency and extremely low-frequency ranges, can be two-fold in nature. On the one hand, the negative effect of magnetic fields is associated with the need to protect the human body since its effect on living systems can lead to DNA damage: it can initiate oxidative modification of nitrogenous bases and increase the number of single- and double-strand breaks [22, 27, 28]. On the other hand, in recent decades, extremely low-frequency MFs have occupied a special place in medical research, especially in the field of cancer treatment and in combination with chemotherapeutic drugs and pain relief [29, 30]. It is assumed that the rate of diffusion through biological membranes, the orientation and conformation of biological macromolecules, and the concentration of free radicals can all change under the action of MFs [22, 31, 32]. In neurophysiology, transcranial magnetic stimulation (TMS)—technology that stimulates the brain and cortical neurons using alternating magnetic fields—is increasingly being used [33]. Studies, where TMS therapy is combined with genetics, have clearly indicated that the genome is the main factor that controls the effects of TMS (neuronal susceptibility and exposure strength) [34, 35]. In addition, in recent studies, the presence of natural oscillation frequencies in genes was established, depending on the nucleotide sequence, which may be the reason for the presence of individual frequencies that cause biological effects [36]. In our opinion, one of the objectives of research being conducted in this area should be to study the effect of gene polymorphisms and their sensitivity to low-intensity, variable MFs, which are used in medical practices.
Currently, there are no generally accepted hypotheses in the field of low-frequency MF that depict how they influence biological systems or theoretical models describing the mechanisms of these influences [37]. However, detailed physicochemical processes that determine the parameters of biochemical reactions and form the body’s response to external influences are not taken into account in theoretical models [19, 21, 22]. Low-frequency MFs cannot cause thermal effects directly and make significant changes in the rate constants of biochemical reactions due to temperature effects. We believe that these fields can act indirectly by changing the concentrations of some kinetically significant molecules, such as ROS.
In this work, the 8-oxoguanine-DNA-glycosylase (hOGG1) gene polymorphism was studied, and the nature of oxidative damage to DNA was revealed before and after exposure to an alternating magnetic field in vitro by determining the level of 8-oxoG in DNA in groups of donors with different polymorphisms. A model of the low-frequency magnetic field action mechanism on aqueous solutions of nucleic acids and proteins has been developed.
For an experimental, non-randomized study, donor blood samples were acquired and genomic DNA was isolated. The study involved 93, apparently, healthy donors, including men aged 21–23 years, who were university students and, according to the survey, did not have any previous pathologies or diseases. Donors provided written consent to participate in the experiment. Blood was taken from the cubital vein and placed into test tubes with an EDTA anticoagulant.
DNA from bioassays was isolated using reagents from ready-made commercial
AmpliSense kits—“DNA-Extran-1” (Cat. EX-509, FSUE TSNIIE Rospotrebnadzor,
Moscow, Russia)—using the sorption method [38]. The DNA concentration in the
final solution was determined spectrophotometrically, the extinction coefficient
was E
Typing of the rs1052133 polymorphic variant of the 8-oxoguanin DNA glycosylase (hOGG1) gene was carried out by real-time polymerase chain reaction using TaqMan probes on a Rotor-Gene Q amplifier (S/N: R0812156, Qiagen, Germany), with reagents for determining the polymorphism of the 8-oxoguanin DNA glycosylase gene (rs1052133) (Cat.No.NP-465-100, Syntol LLC, Moscow, Russia). The reaction mixture for determining the Pro332Ala polymorphism of the 8-oxoguanin DNA glycosylase (hOGG1) gene (rs1052133) was prepared, according to the protocol from 32 test samples plus 4 additional samples (3 positive and 1 negative sample).
Using the Rotor-Gene Q software (version 2.3.5, Qiagen, Germany), the temperature regimes, the duration of each cycle, and the number of repetitions in each stage were set; their values are shown in Table 1.
PCR steps | Temperature, °C | Duration, sec | Number of cycles |
Melting | 94 | 180 | 1 |
Cycling 1 | 94 | 20 | 10 |
58 | 20 | ||
61 | 30 | ||
Cycling 2 | 94 | 20 | 30 |
58 | 20 | ||
61 | 30 |
After the amplification was completed, the results of the study were analyzed using the Rotor-Gene Q software (version 2.3.5, Qiagen, Germany), with the threshold line set to 0.1, and the areas of the corresponding genotypes were highlighted (i.e., define genotype, wild type, heterozygous, and mutant). The results were interpreted according to Table 2.
Allele 1 (green) | Allele С | Genotype С/С |
Heterozygote (green + yellow) | Allele С + Allele G | Genotype С/G |
Allele 2 (yellow) | Allele G | Genotype G/G |
The flanking sequence was considered, and the frequency of the minor allele G was taken as equal to 30.21%, which corresponded to 1000 genomes.
The correspondence of the distribution of genotypes to the Hardy–Weinberg
equilibrium was assessed using the WpCalc online service
(https://wpcalc.com/en/equilibrium-hardy-weinberg/). Criterion
The level of 8-oxyguanine in the DNA was determined by enzyme immunoassay using monoclonal antibodies to 8-oxoG with DNA damage—kit (determination of 8-OH-2-deoxyguanosine, catalog number EC-360, Immun diagnostics, Berlin, Germany), according to the protocol. Antibody solutions to 8-oxoG were preliminarily prepared at a dilution of 1:250 and at a dilution of 1:500 for the conjugated mouse IgG antibodies. A total of 50 µL of 8-oxoG antibodies were added to the wells, incubated for 1 hour, and washed 6 times with wash buffer. Next, 50 µL of mouse IgG antibody conjugate was added, similarly incubated and washed. Then, 50 µL of tetramethylbenzidine substrate (chromogen) was introduced and after instant coloring, the mixture was incubated for 15 min in the dark, and the reaction was completed by adding the stop solution. After the addition of the stop solution, the optical density of the samples was measured at a wavelength of 450 nm using a Thermo Fisher Scientific Multiskan FC microplate reader (Type: 357; S/N: 357-902375, Thermo Scientific, MA, USA). In each experiment, measurements were recorded at least three times, and the average value was determined. The content of 8-oxoG DNA was quantified using a prebuilt calibration curve, which was linear over the 8-oxoG concentration range of 0.94–60 ng/mL. The sensitivity was 0.59 ng/mL.
The samples were treated with an alternating magnetic field using a device developed by us for the automated study of biological fluids, a detailed description and structural diagram of the device is provided in [38, 39]. The scheme of the device is shown in Fig. 1.
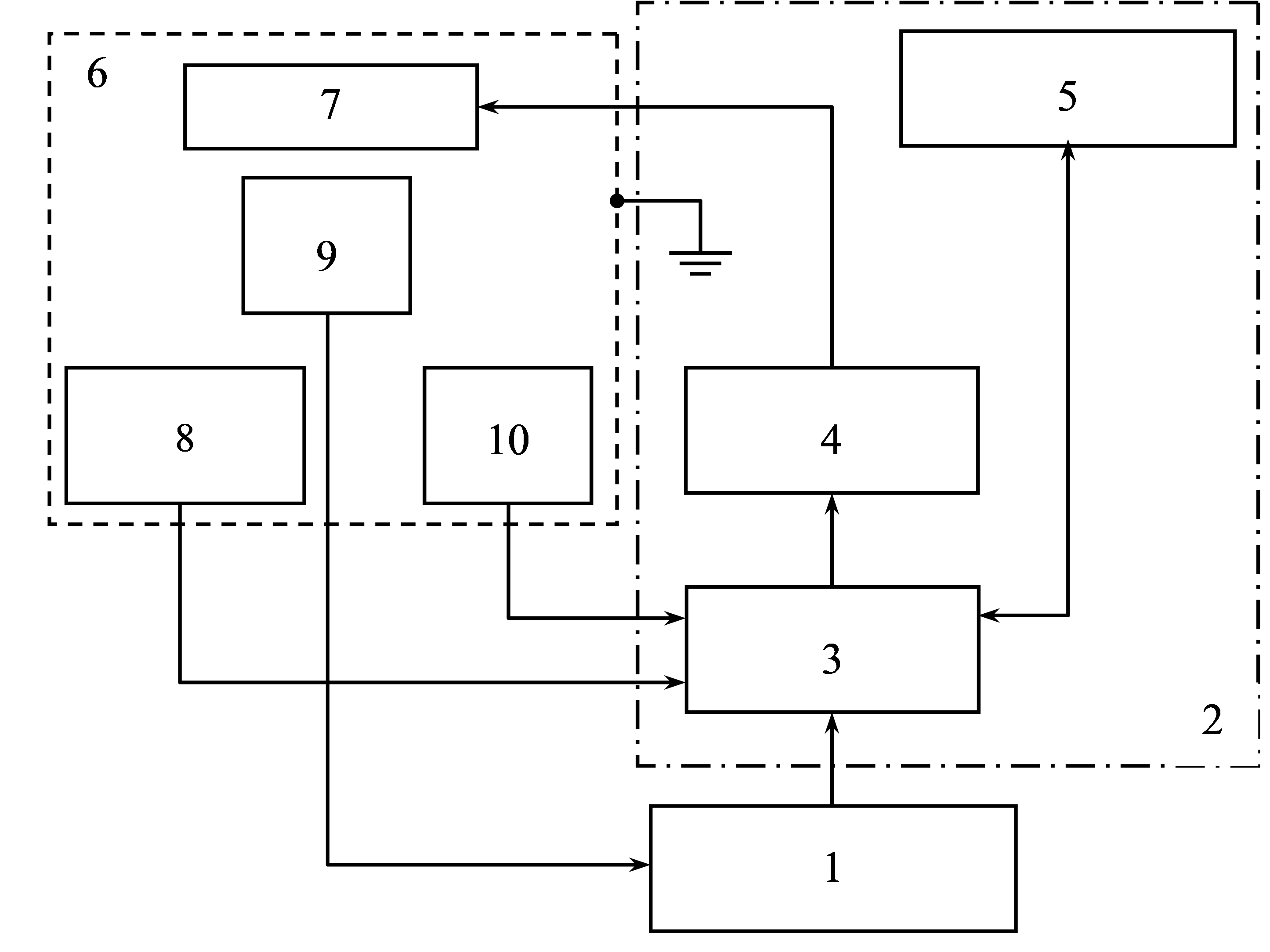
Structural diagram of a device for automated search for optimal parameters for processing biological fluids in an alternating magnetic field. 1: personal computer; 2: generator unit; 3: microcontroller; 4: power amplifier; 5: control and indication unit; 6: grounded shielded chamber; 7: emitter; 8: electromagnetic field strength sensor; 9: measuring module with a sample; 10: temperature sensor.
To increase the homogeneity of the alternating magnetic field during the
processing of biological objects, an emitter 7 was manufactured in the form of a
solenoid. The coil resistance was 20 ohms; wire type PEV-1, wire diameter with
insulation of 0.81 mm; 2735 coil turns; 12 layers; wire length of 485.309 m; coil
inductance of 84 mH. The coil was placed in a shielded chamber 6, which was made
of structural steel, 3 mm in thickness. The weakening of the external
electromagnetic field by the chamber in the range from 3 Hz to 300 kHz reached
100 times. The constant magnetic field (geomagnetic field) was measured using an
Aktakom ATT-8701 magnetic induction meter (Russia). The magnitude of the magnetic
field strength in the open space was 40.00
The hydrogen peroxide content in the aqueous DNA solutions was determined using
the spectrophotometric method, while the PerOx method was used to determine the
peroxides in the plasma EDTA samples (Immun Diagnostic AG, Germany), using a
Thermo Fisher Scientific Multiskan FC microplate reader (Type: 357; S/N:
357-902375, Thermo Scientific, MA, USA). The calibration graph is
linear in the range 1
The test the hypothesis and determine if the data followed a normal (Gaussian)
type of distribution the Shapiro–Wilks test was used. Additionally, the
ordinates of the normalized Gaussian distribution function were determined
according to the recommendations outlined in the monograph [40]. The significance
of the degree of oxidative damage to DNA was assessed by the nonparametric
Mann–Whitney U-test. Differences in all studied parameters were considered
statistically significant at p
The study was approved by the Independent Ethics Committee of the Federal State Budgetary Educational Institution of Higher Education “Kuban State Medical University” of the Ministry of Health of the Russian Federation (4 Mitrofan Sedin St., Krasnodar, Russia), protocol No. 89 of June 26, 2020.
When analyzing the polymorphism of the 8-oxoguanine-DNA-glycosylase (hOGG1) gene in healthy donors, genotypes were divided into homozygotes (wild type), heterozygotes, and homozygotes for the recessive allele (mutant type).
The distribution of genotypes for the rs1052133 locus in the hOGG1 gene is shown
in Table 3. As can be seen from Table 3, in healthy donors, the homozygous CC
genotype of the hOGG1 gene was mainly observed; however, heterozygous CG and
homozygous GG genotypes were also observed. From the data presented in Table 3,
it can be seen that in the control group of conditionally healthy donors, the CC
genotype is predominant, with it identified in 68 people, which is 73% of the
participants. The CG genotype accounts for 25% (23 people) and the GG genotype
for 2% (2 people). From the ratio of allele frequencies, calculated according to
the Hardy–Weinberg equation, it was found that the differences in the frequency
of the C allele between the two groups are not significant: this indicator in the
first group was 0.94, while in the second it was 0.83. The G allele frequency in
the second group of donors was 0.15, which is significantly higher than in the
first group, which demonstrated an allele frequency of 0.05 (p
Genotypes, alleles | Group, n = 93 people |
abs, % | |
СС | 68 |
73% | |
СG | 23* |
25%* | |
GG | 2 |
2% | |
С | 0.85 |
G | 0.15 |
*df = 2;
According to the results of the PCR analysis, the donors were divided into two groups: those with the C/C genotype hOGG1 (68 people; group 1) and C/G and G/G genotypes (25 people; group 2). The accumulation of 8-oxoG in the blood serum and a decrease in the stability of the genetic material with an increasing oxidative load can act as an indicator of genome destabilization.
To determine the resistance of the genetic material to external influences, further studies were carried out on the effects of an alternating magnetic field (MF) on the appearance of oxidative DNA damage in conditionally healthy donors.
The degree of oxidative DNA damage was assessed by changes in the concentration
of 8-oxoG in the blood serum of donors. The concentrations of 8-oxoG in DNA from
the blood serum of healthy donors in the first and second treatment groups are
shown in Fig. 2, for both before and after the in vitro treatment of
blood samples with a magnetic field, at frequencies of 3, 30, and 50 Hz. These
frequencies were selected based on previous studies [21, 41]. The initial level
in the control samples, without exposure to the field, was 7.4
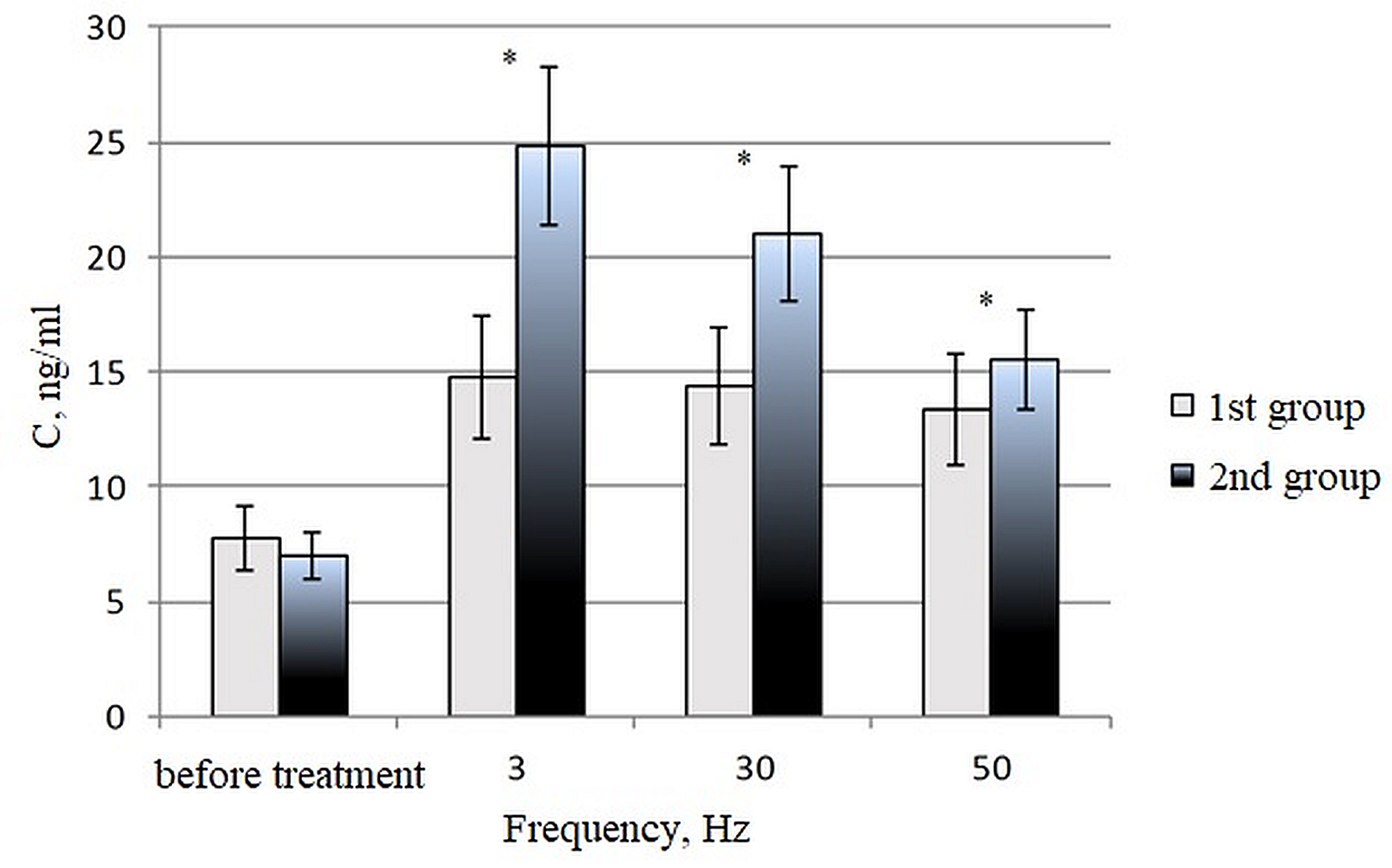
The 8-oxoG content in the blood serum of healthy donors in the
first group (C/C polymorphism of the hOGG1 gene) and the second group (C/G and
G/G polymorphisms of the hOGG1 gene) after treating blood samples in
vitro with a magnetic field at frequencies of 3, 30 and 50 Hz for 30 min. U =
1.5; U critical = 3 for p
As noted in a number of studies [6, 7, 8, 9, 10, 11, 12, 13, 14, 19, 20, 23], magnetic fields affect animals and cells by promoting the generation of reactive oxygen species (ROS), potentially leading to oxidative stress at the cellular and systemic levels. An increased level of ROS generation, mediated by physical, chemical, or biological environmental factors, is the most genotoxic process affecting DNA [20, 22, 23].
To study the mechanisms involved in the process of magnetic fields causing the formation of free radicals in the blood, we developed a mathematical model. However, to simplify calculations, the model was used to study an aqueous solution of DNA isolated from the blood of apparently healthy donors.
In dilute solutions of biopolymers, the processes of ROS formation proceed as a chemical oscillator [42]. To describe the chemical oscillator in the conversion of hydrogen peroxide, the longest-lived ROS in an aqueous solution, we used chemical equations with known simplifications.
The initial stage is the initiation of the process, i.e., the attachment of an electron from e-c Rydberg excited levels of macromolecules (for example, DNA) [43], to free protons of water, to the formation of a hydrogen radical:
at neutral pH, the hydrogen radical interacts with dissolved oxygen molecules,
the concentration of which is 3 orders of magnitude higher than the concentration
of protons and hydroxide ions (10
further formation of peroxide occurs in two ways:
or
as the concentration of hydrogen peroxide increases, according to Eqns. 3–4, the pH of the solution increases. When the aqueous environment is at a neutral and alkaline pH, hydroperoxide radicals decompose to form superoxide ions:
the released proton can again interact with the Rydberg e-
the presence of a singlet oxygen in a neutral and weakly alkaline medium leads to the further formation of superoxide ions:
dismutation of the hydroxyl radical leads to the formation of hydrogen peroxide:
where the hydroxyl radical can interact with the resulting hydrogen peroxide:
As the pH increases, peroxide is able to spontaneously decompose with the acidification of the medium:
or with the formation of singlet oxygen:
self-decomposition of peroxide, according to for the rate constants of Eqn. 10, has an order of
magnitude from 1 to 2 and leads to the formation of singlet oxygen
By introducing the notation k
from Eqn. 12, it follows that in dilute solutions of biopolymers, the rate
of accumulation of hydrogen peroxide depends on the pH of the solution, the
concentration of electrons
Let us consider the formation of a chemical oscillator involved in ROS
interconversions in aqueous solutions of biopolymers. Fig. 3 shows a simplified
schematic of H
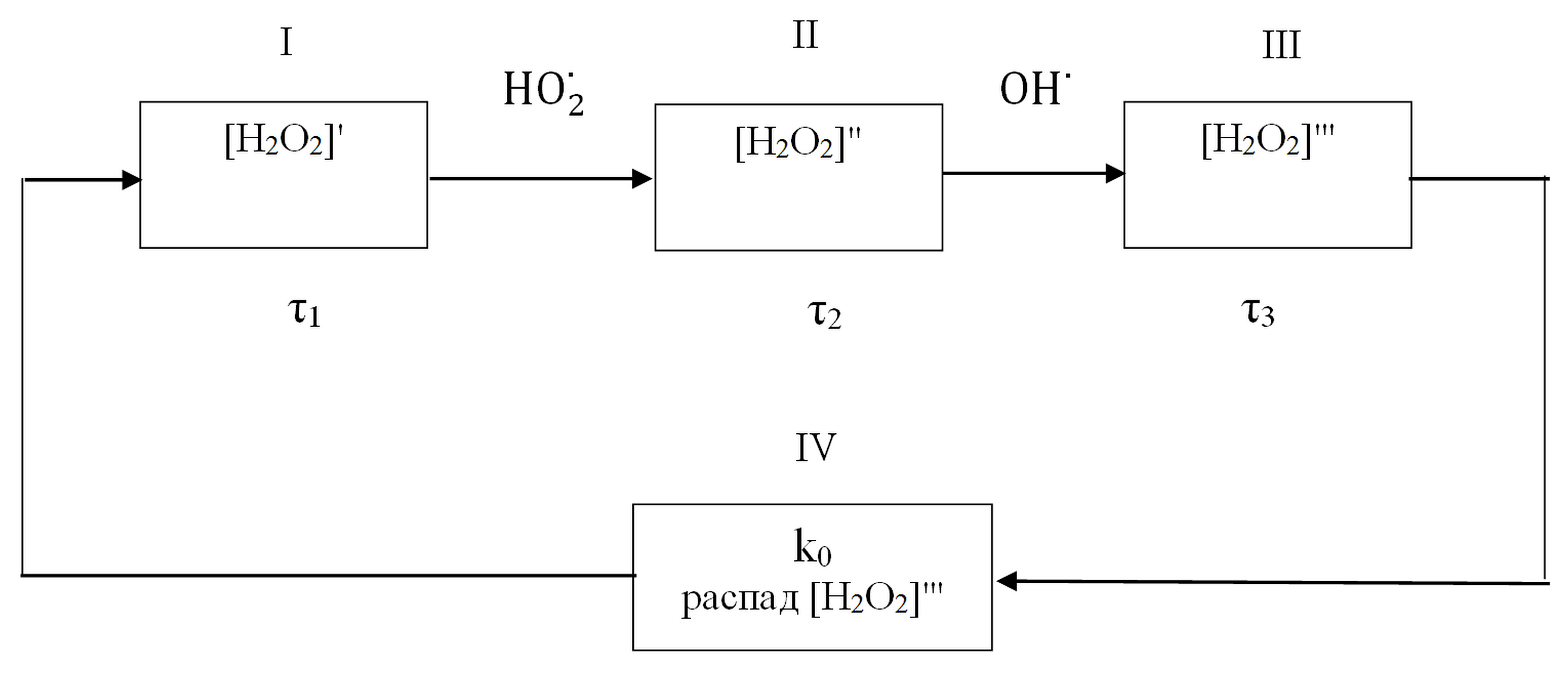
Schematic of interconversions of hydrogen peroxide in a biopolymer aqueous solution for reactions (1)–(11).
Let us introduce the value
taking into account the occurrence of hydrogen peroxide decomposition reactions
and a decrease in its concentration, the index k
Eqn. 17 is a differential equation for free oscillations that arise in a
harmonic oscillator [47]. The expression in front of [H
and the expression before
Then, Eqn. 17 will take the form:
Let us consider the influence of a low-intensity and low-frequency magnetic
field, which sine changes and is described by the equation
H = H
According to Eqn. 12, in biopolymer solutions, the rate of hydrogen
peroxide accumulation depends on, among other things, the concentration of
electrons at the Rydberg excited levels of macromolecules. A low-intensity
magnetic field can affect the magnetic moments of these electrons. In addition,
in aqueous solutions of biopolymers, there are radical pairs with different
g-factors of partners (for example,
All of the above suggests that under the influence of a magnetic field, the increment in the concentration of hydrogen peroxide changes in proportion to its strength:
where
As a result, the oscillations arising in the chemical oscillator will become forced, causing the following equation to become valid for them:
the solution of such an inhomogeneous equation is equal to the sum of the general solution of a homogeneous equation that characterizes the decaying process of hydrogen peroxide formation, which occurs after the application of an external influence, as well as a particular solution that describes a steady oscillatory process under the action of an applied driving force.
If the losses in the chemical oscillator are small, and the frequency of the driving force differs slightly from the natural frequency of the oscillations in the hydrogen peroxide concentration in the chemical oscillator, then, we can obtain the solution for Eqn. 19 in the following form:
additionally, the value of the amplitude of the forced fluctuations in the concentration of hydrogen peroxide under the action of an alternating MF is described by the expression:
thus, it follows from Eqn. 20 that natural oscillations in the hydrogen
peroxide concentration decay with time in the chemical oscillator, and only
forced oscillations in the H
Theoretical dependences for the content of hydrogen peroxide in an aqueous solution of DNA relying on the frequency of an alternating magnetic field have been constructed. The calculation considered the strength of the magnetic field –450 A/m and the attenuation index of the fluctuations in the hydrogen peroxide concentrations in the chemical oscillator was 0.7–2.5 (Fig. 4).
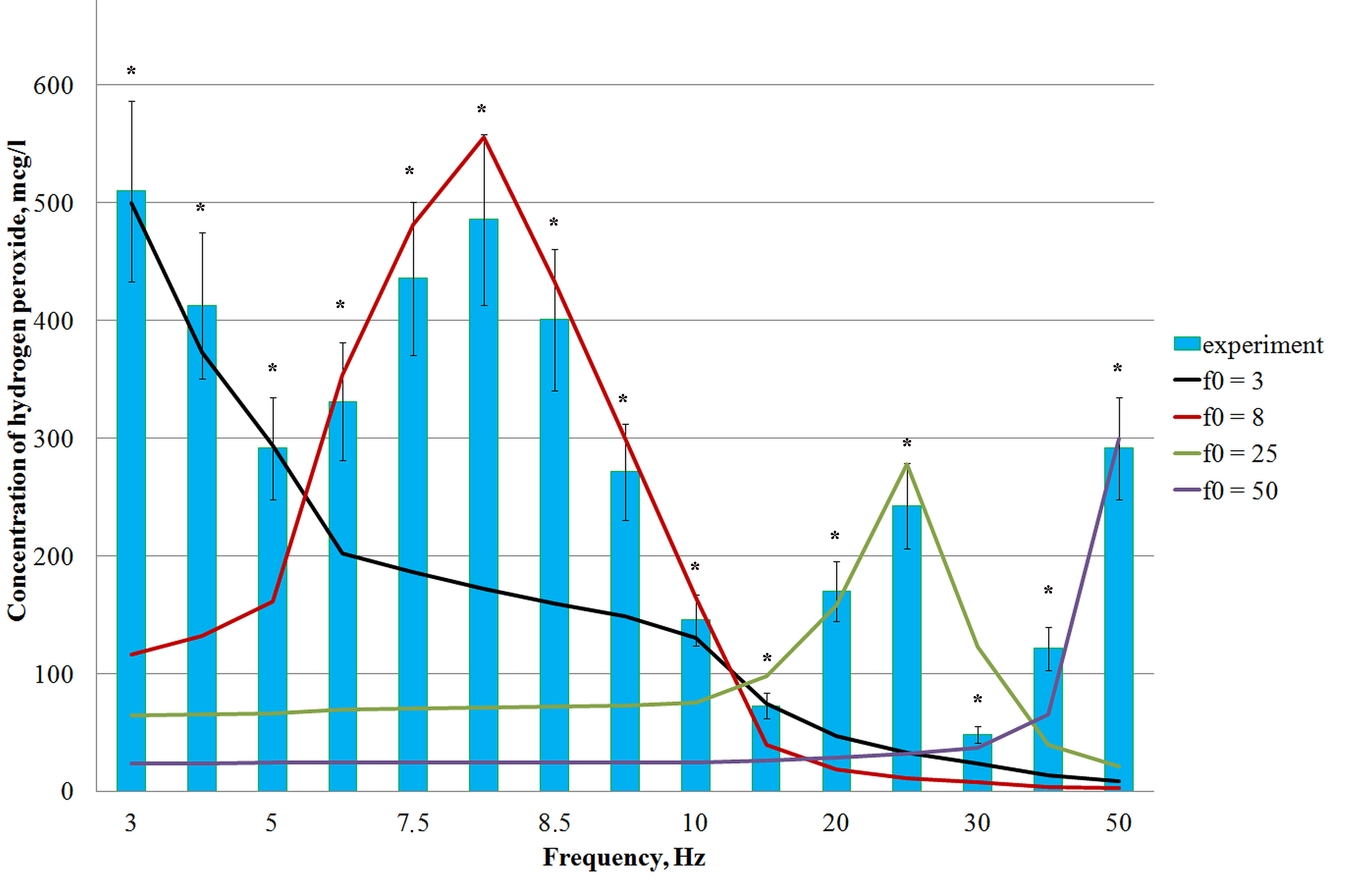
The content of hydrogen peroxide in an aqueous solution of DNA
depends on the frequency of the magnetic field, calculated by Eqn. 21, and
the experimentally determined content of hydrogen peroxide in aqueous solutions
of DNA with a concentration of 0.025 µg/mL after exposure to different
magnetic field frequencies at a temperature of 21 °C and
tension of 450 A/m. The exposure time for each solution was 30 min. *:
statistically significant differences compared with the control (samples not
treated with a magnetic field) at p
To verify the model, the theoretically calculated and experimentally obtained
values for the hydrogen peroxide concentrations were compared after treatment of
aqueous DNA solutions with a low-intensity MF at the corresponding frequencies.
The results are shown in Fig. 5. The H
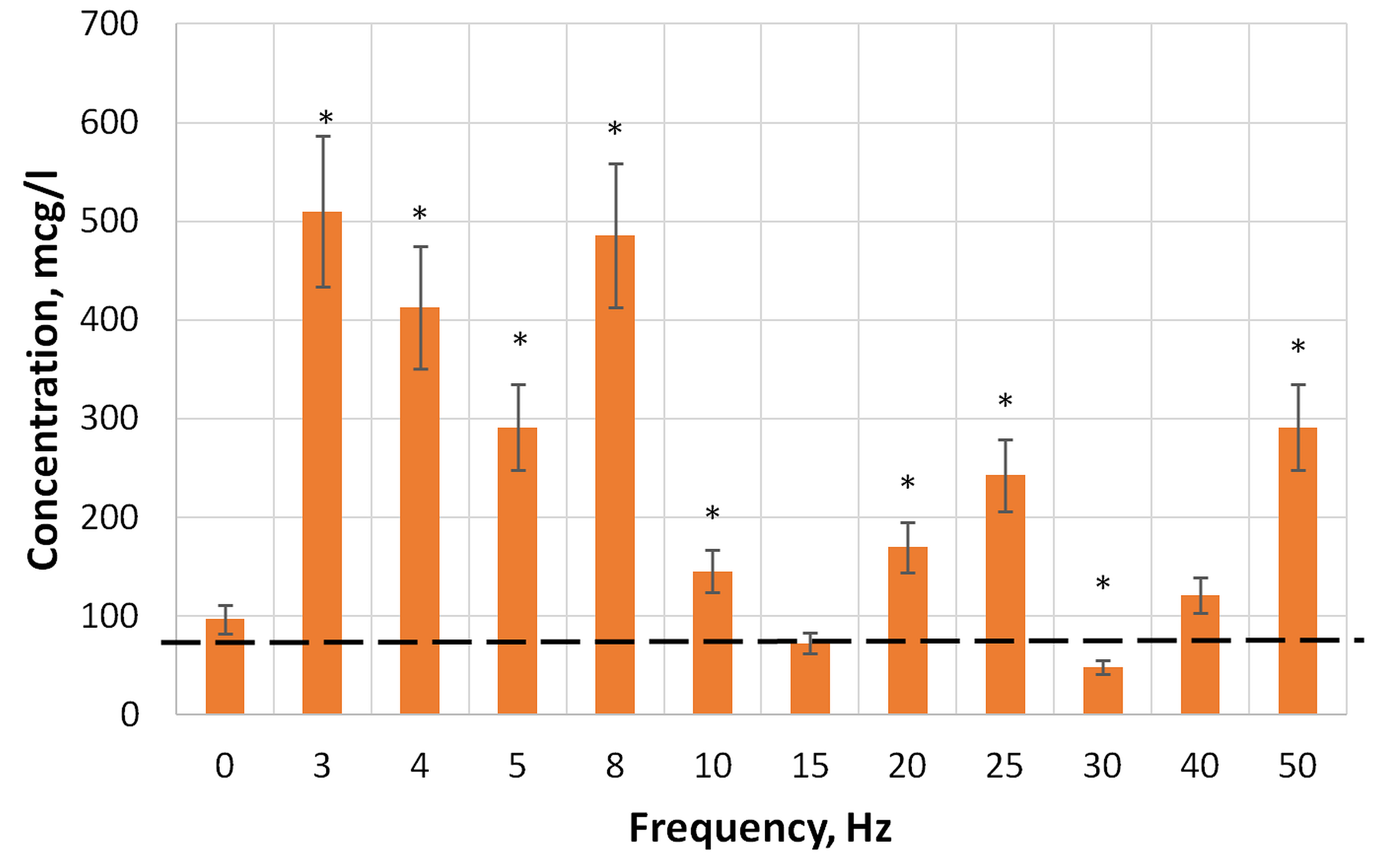
The content of hydrogen peroxide in aqueous solutions of DNA
with a concentration of 0.025 µg/mL, before (0 Hz) and after
exposure to magnetic fields of different frequencies at 21 °C, with an
exposure time for each solution of 30 min.*: statistically significant
differences compared with the control (samples not treated with a magnetic field)
at p
The kinetic mechanism of interaction between the enzyme 8-oxoguanine DNA glycosylase hOGG1 and the damaged site in DNA consists of several stages [21]. The first step in the interaction between the hOGG1 enzyme and DNA is the formation of a nonspecific complex. The process of primary binding of the oxoG substrate leads to the rapid inversion of 8-oxoG from the double helix, first into the “precatalytic”, and then, into the catalytic center of the enzyme. In the second stage of the binding process, the formed cavity in the DNA duplex is filled with specific amino acid residues and the ribose phosphate backbone of the substrate begins to bend. However, as was shown using the 8-oxoG–aPu substrate, the third stage also reflects the process of the incorporation of amino acid residues into the DNA duplex. Only in the third complex is the bending of the duplex completed and a complete system of contacts is formed between the enzyme and DNA substrate, which is necessary for the catalytic stage of the process to proceed [21]. Apparently, the functioning of the enzyme 8-oxoguanine DNA glycosylase hOGG1 in C/G and G/G polymorphisms of the 8-oxoguanine DNA glycosylase (hOGG1) gene is sharply reduced, which does not allow for the restoration of the DNA structure. Conversely, the accumulation of 8-oxoG in DNA, which occurs upon exposure to a low-frequency MF, can lead to the appearance of new mutations, including those that generally weaken the processes of reparative regeneration.
DNA-binding proteins protect chromosomes from oxidative damage. This is due both to the compact packaging of the DNA molecule and the interaction of proteins with the resulting ROS. However, it was found in [48], that long-lived protein radicals are sources of long-term ROS formation and are capable of transferring radical damage to DNA, for example, by histone H1 hydroperoxides, which can induce the formation of 8-oxoguanine in DNA [49]. All ROS and protein radicals, which are formed upon the exposure of blood samples to low-intensity MFs, can attack the DNA molecule, thereby leading to oxidative damage of nitrogenous bases and the accumulation of 8-oxoG in DNA.
This conclusion is in accordance with the results of previous work by Blank and Goodman, who found DNA sequences in the promoters of the c-myc and HSP70 genes, which they called response elements to electromagnetic field exposure [50]. They argue that the activation of these genes, which depends on the impact of an electromagnetic field, is associated with the destabilization of hydrogen bonds in these response DNA elements under the influence of the field [51]. A number of researchers believe that MFs affect biological systems by enhancing (or creating) ROS [23, 24]. ROS are known to cause a wide variety of biological consequences, including DNA damage and the possible induction of mutations. Simko and Mattsson presented some evidence indicating that exposure to extremely low-frequency MFs increases free radical levels in cells through several mechanisms, yet mainly through the activation of macrophages (or other cell types that produce ROS) [52]. It is known that the body has developed a multilevel system of protection to repair any damage to DNA that occurs under the action of ROS. Obviously, DNA damage repair resulting from ROS requires not only antioxidant defense enzymes but also excision repair enzymes [53, 54, 55]. To describe the possible mechanism of action in biological systems following exposure to a low-frequency MF, we have developed a model that considers changes in hydrogen peroxide concentration in aqueous media under the action of an MF.
Using an example of an aqueous solution of DNA with a known concentration, the
theoretical dependences of the hydrogen peroxide content are compared to the
experimental results. Fig. 4 shows the theoretically calculated dependences of
the hydrogen peroxide content on the frequency of a low-intensity magnetic field
(solid lines), using Eqn. 21, and the experimentally determined
concentrations of hydrogen peroxide in aqueous DNA solutions with a concentration
of 0.025 µg/mL after their exposure to an MF with the corresponding
frequencies (bars). It can be seen that each theoretical dependency presents a
maximum concentration of hydrogen peroxide in the solution, while four
dependencies were identified in the frequency range from 3 to 50 Hz: at
frequencies (f) of 3, 8, 25, and 50 Hz. Each maximum H
A change in the hydrogen peroxide concentration in an aqueous solution of biopolymers makes it possible to explain the observed effects as a result of the influence of the low-frequency alternating MF on biochemical processes. Thus, a significant increase in the content of hydrogen peroxide in DNA solutions following exposure to alternating magnetic fields with frequencies of 3–8 and 50 Hz (Fig. 5) leads to an increase in the level of oxidatively modified nitrogenous DNA bases. This is interesting since a significant (1.5 times) increase in the level of 8-hydroxy-2-deoxyguanosine in genomic DNA is a key biomarker of oxidative damage to nucleic acids mediated by ROS generation.
Considering that H
The proposed model of the mechanism of action of a low-intensity alternating magnetic field on aqueous solutions of biopolymers does not consider the biophysical nature of the target and takes into account only the nature of its interaction with the aqueous environment. Despite the fact that water is considered stable and relatively inert, reports of its exceptional behavior have attracted considerable interest recently. Thus, water molecules are spontaneously oxidized with the formation of hydrogen peroxide near the water-air interface of micron-sized water droplets [61, 62]. This process does not require any chemical reagent, catalyst, applied electrical potential, or radiation, and is associated, according to the authors, with the presence of a superstrong electric field at the air-water interface. Similar phenomena were also observed by the authors of [63], although they explained them not by the interfacial effect of drops, but by ultrasonic treatment. Thus, water should be considered as a non-equilibrium active dynamic system, which is very sensitive to weak external influences of physical (low-intensity and low-frequency MF) and chemical (presence of a biopolymer) nature. On the whole, the processes of ROS formation in the aquatic environment, which occur like a chemical oscillator, gradually fade if not supported by external energy of low-intensity factors.
Under the action of a low-intensity and low-frequency MF on aqueous solutions of biopolymers, a significant role is played by collective dynamic and structural rearrangements, in which ROS are both regulatory and control elements. As the authors of the works [64, 65] found, periodic rhythms involving ROS depend on fluctuations in the external field as a control (or modulating) signal. Under normal physiological conditions, the production of ROS and the action of chemical oscillators on fluctuations in the concentration of hydrogen peroxide occur rhythmically, while all other cellular processes are balanced and synchronized. The impact of low-intensity MFs affecting the pro-oxidant–antioxidant system in the body, changes with the intensity of free-radical oxidations and the potential of the endogenous antioxidant system of the body.
Thus, although the action of an MF does not directly affect DNA, under some circumstances it produces a biological effect reminiscent of heat shock and/or stress [37]. This effect is mild and depends on the state of cellular homeostasis prior to exposure and the polymorphism of genes that are sensitive to the low-intensity variable MF being emitted.
Donors with C/G and G/G polymorphisms in the 8-oxoguanin DNA glycosylase (hOGG1) gene are more sensitive to the effects of low-frequency magnetic fields, while donors with a C/C polymorphic hOGG1 are much less susceptible to the actions of low-frequency MFs, as evidenced by the elevated levels of 8-oxoG in the blood serum of donors in the second group after the in vitro treatment of their blood samples with MF, compared with the first group.
A model has been proposed for the mechanism of action by low-frequency magnetic fields, with extremely low energies, on aqueous solutions of biopolymers containing nucleic acids and proteins. The key element in this concept is the chemical oscillators of ROS generation in the aqueous medium, in which competition by the physical and chemical processes (electron transfers, reactions of decay and addition of radicals, spin magnetically induced conversion, synthesis, and decay of hydrogen peroxide) is controlled by the magnetic field. Changes in the concentration of hydrogen peroxide in aqueous solutions of biopolymers make it possible to explain the experimentally observed effects of the low-frequency magnetic field on the biochemical processes. The resulting increased ROS attacks on the nucleophilic centers of proteins and DNA molecules, ultimately, change their states. Thus, damage to biopolymers in aqueous solutions resulting from exposure to low-intensity magnetic fields is similar to damage that occurs during oxidative stress.
The datasets used and/or analyzed during the current study are available from the corresponding author on reasonable request.
ET, MB, SD, AD, VM and GI jointly conceptualized, searched available literature, wrote the original draft and edited the final draft. All authors read and approved the final manuscript. All authors have participated sufficiently in the work to take public responsibility for appropriate portions of the content and agreed to be accountable for all aspects of the work in ensuring that questions related to its accuracy or integrity.
The study was approved by the Independent Ethics Committee of the Federal State Budgetary Educational Institution of Higher Education “Kuban State Medical University” of the Ministry of Health of the Russian Federation (4 Mitrofan Sedin St., Krasnodar, Russia), protocol No. 89 of June 26, 2020.
Not applicable.
This work was financially supported by the state assignment of the Ministry of Education and Science to the Kuban State University FZEN-2023-0006.
Given their role as Guest Editors, Anna Dorohova and Stepan Dzhimak had no involvement in the peer-review of this article and has no access to information regarding its peer-review. Full responsibility for the editorial process for this article was delegated to Amedeo Amedei.
Publisher’s Note: IMR Press stays neutral with regard to jurisdictional claims in published maps and institutional affiliations.