- Academic Editor
Biodegradation and biotransformation of contaminants in groundwater commonly occurs naturally. However, natural biodegradation rates can be slow leading to elongated contaminant plumes and prolonged risks that demand greater remedial intervention. Enhancement of the biodegradation of contaminants in groundwater can be induced by the addition of amendments to change the geochemical conditions to those that are more favorable for indigenous or added biota. Enhancing biodegradation requires collocation of the contaminant of concern with the ‘right’ microbial communities under the ‘right’ geochemical conditions, so that the microbiota thrive and bio-transform, degrade or lock up the contaminant of interest. This is most easily achievable at laboratory or bench scale where mixing is easily performed, and mass transfer limitations are minimized. However, inducing such changes at field scale in aquifers is non-trivial - amendments do not easily mix into groundwater because it is a laminar (non-turbulent) and low-energy flow environment. Bioaugmentation of cultured or genetically modified organisms have also been considered to add to groundwater to enhance contaminant degradation rates. Here we provide an overview of research studies over approximately 40 years that highlight the progression of understanding from natural biodegradation of plumes in groundwater to active bioremediation efforts that have been variably successful at field scale. Investigated contaminants providing insights include petroleum hydrocarbons, chlorinated and brominated hydrocarbons, ammonium, metals, munition compounds, atrazine and per- and polyfluorinated alkyl substances. The redox and electron acceptor/donor conditions that are inducive to biodegradation for a range of contaminants are highlighted. Biodegradation is challenged by the availability of electron donors/acceptors in the core of plumes and on plume fringes. Cases for bioaugmentation are identified. A long history of investigations provides examples of the importance of amendment delivery mechanisms, scale-up from laboratory to field, and field-scale demonstration of the effectiveness of groundwater bioremediation technologies. Advantages and disadvantages of remedial approaches are tabulated. The value and contributions of integrative modelling advances are identified. The literature review and example cases provide a deep understanding of what scale of bioremediation might be achievable for groundwater plumes. Limitations to bioremediation strategies outlined here will help direct future efforts. Addressing the sources of groundwater plumes as well as bioremediation of the plume itself will achieve more effective outcomes. Twelve ‘lessons learnt’ are synthesized from the review.
A range of chemicals pollute groundwater. From agriculture, nutrients and pesticides (e.g., atrazine) commonly cause dispersed impacts and widespread concerns for groundwater quality [1]. Urban development has been shown to degrade groundwater quality globally with many sources of contaminant releases into groundwater in urban areas from, for example, septage [2], landfills [3], chemical handling (e.g., dry cleaners, weed control) [4, 5, 6], cemeteries [7], disturbance of acid sulfate soils [8], and fuel retailing [9, 10]. From the oil and gas and manufacturing industry petroleum, chlorinated and brominated hydrocarbon contaminants arise and pose some of the most common groundwater contaminants globally [11, 12]. From Defense activities, explosive ordinance (e.g., nitrotoluene explosives, perchlorate) and other chemicals have impacted groundwater quality [13, 14]. Disturbance of sulfide rich sediments and ores during mining often lead to acid generation that leaches metals into groundwater [15, 16]. Recently per- and polyfluorinated alkyl substances (PFAS) have become a regulatory and research focus because of their unusual properties (e.g., partitioning to air-water interfaces in unsaturated source soils), as well as their apparent widespread occurrence and pollution of groundwater and for being highly stable and recalcitrant to degradation [17, 18, 19]. Among these, PFAS and petroleum fuels are highly complex mixtures made up of many thousands of organic compounds with variable properties (e.g., solubility, volatility, density, sorption, degradability) [20, 21, 22] posing particular risks and management challenges.
Many of these contaminants biodegrade, bio-transform and/or can be immobilized (precipitated) via microbially mediated processes. This has been commonly shown to be the case in laboratory settings where often batch and column scale experiments are controlled, and amendments are added to ensure ideal microbial growth conditions, and to provide electron acceptors or donors needed for key bioreactions [23, 24]. Field investigations have created greater confidence in field-scale biodegradation rates, and the potential to harness it for engineered bioremediation of groundwater plumes. In contrast, investigations on PFAS biodegradation are few. PFAS precursors (poly fluorinated PFAS) can bio-transform but commonly do so to perfluorinated PFAS or perfluoroalkyl acids (PFAAs) such as perfluorooctane sulfonic acid (PFOS). However, PFAAs have not been shown to degrade or otherwise transform under ambient environmental conditions [25, 26, 27].
Here we review fundamental understanding gained from studies of the fate and biodegradation of contaminants in groundwater. Specific examples are taken from a range of organic and inorganic contaminant investigations. Whilst microorganisms can also be harnessed to hydraulically contain groundwater movement via enhanced clogging of aquifers [28], this review will largely focus on degradation, transformation and immobilization processes and their potential and limitations. Both natural biodegradation processes and selected engineered bioremediation approaches are given as examples, along with efforts to better integrate processes occurring in groundwater to predict and understand contaminant behavior when undergoing biodegradation. Earlier references are citated to alert the reader to pioneering work and to give an indication of understanding and contributions at that point in time and the maturation of our knowledge over the last 40 years.
Biodegradation requires collocation of the contaminant of concern with the ‘right’ microorganisms under the ‘right’ geochemical conditions, so that the microorganisms thrive and bio-transform, degrade or lock up the contaminant of interest. Often the ‘right’ geochemical conditions might include redox sensitive species: electron acceptors such as oxygen, nitrate, sulfate, or iron oxides which are typically utilized during the biodegradation of petroleum hydrocarbons for example, or electron donors such as natural organic carbon and soluble carbon sources which are typically utilized during the biodegradation of chlorinated hydrocarbons [29]. Other chemicals that may limit the metabolism of microorganisms might be trace nutrient levels in aquifers. Natural rates of biodegradation may be low where collocation is limited or rates of replenishment or mixing that support the right geochemical and metabolic conditions are slow. Contaminants and other geochemical species might partition to or from the soil matrix, react with soluble or mineral phases and interact along groundwater flow pathways. A pore-scale view of some of the key processes is depicted in Fig. 1.
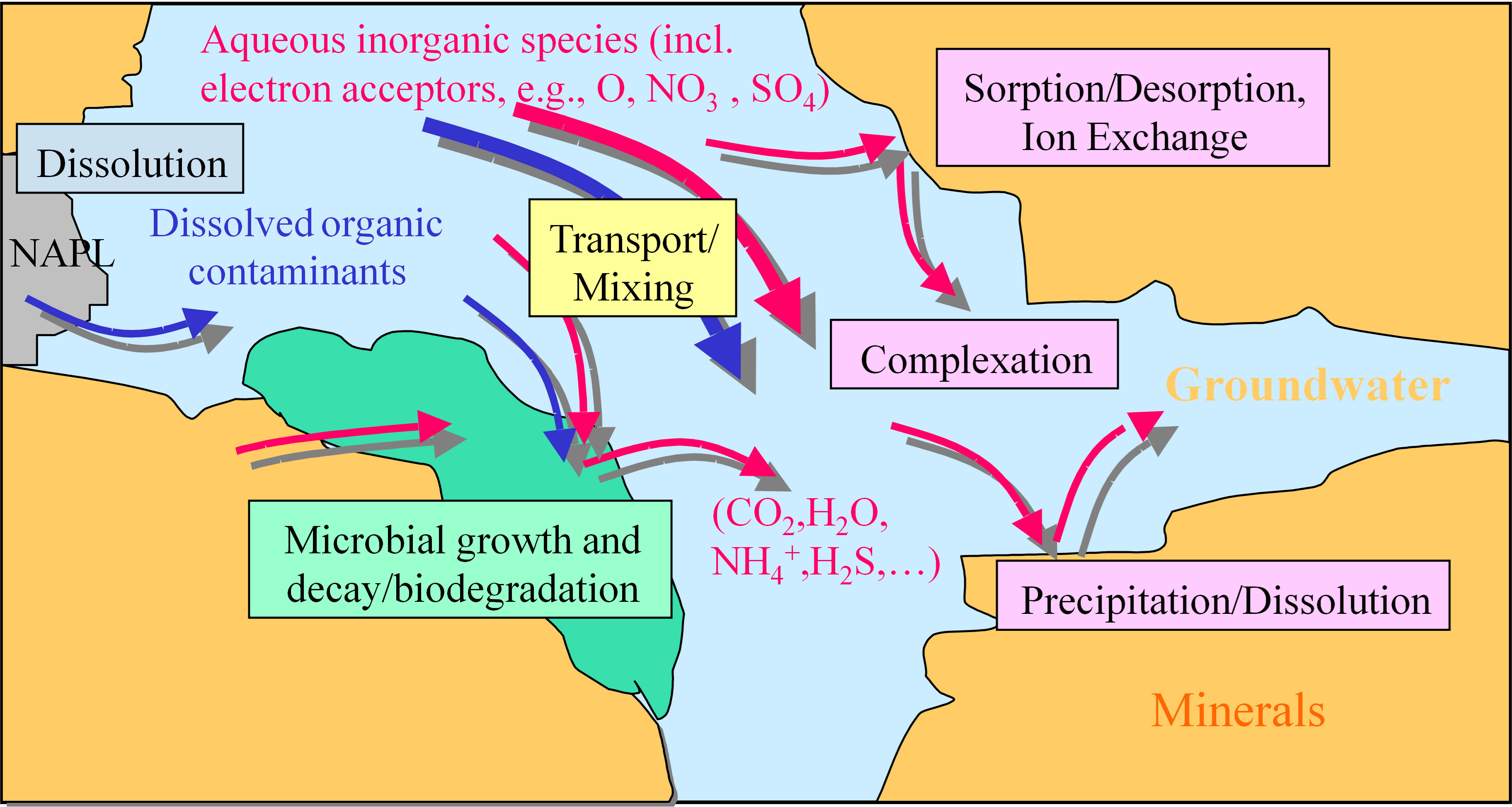
Pore scale view (within a porous aquifer) of a range of processes that may be active in determining the transport, fate and potential biodegradation, partitioning and overall attenuation of contaminants in groundwater.
Microorganisms naturally occur in groundwater and aquifers [30, 31, 32, 33], and studies indicate that a large proportion reside on solid aquifer media surfaces compared to being suspended in the liquid phase [34]. Microorganisms seem able to live in micro-niches that are not overly toxic to themselves, but also contain enough ‘food’ to sustain them. They are able to degrade contaminants that are deemed highly weathered for example highly weathered diesel or crude oil [35], and some are motile with chemotactic mechanisms that allow them access to food sources distant from their immediate location [36]. Recharge to groundwater itself, brings chemical changes that induce groundwater ecosystem changes [37].
Groundwater environments are not well mixed. In the laboratory, mixing is more easily induced to achieve contact between contaminants, microbial communities and amendments that may be needed to create advantageous conditions for microorganisms to induce greater biodegradation [6, 38, 39]. Typically, groundwater flows through the pore spaces in aquifers at low rates, and exhibits laminar, stable and non-turbulent flow [40]. The implication is that as contaminants enter groundwater in infiltrating water from overlying soils they travel along set flow pathways in aquifers, governed by the hydraulic conditions, the pore space and the intrinsic heterogeneity of aquifer strata and properties. As such, limited mixing of adjacent water or dilution of contaminant concentrations occurs except via the scale of velocity variations induced by aquifer heterogeneities; this latter process is commonly termed dispersion [40].
Investigations have shown that dispersion can be small and where the contaminant and relevant electron acceptor/donor are both moving with groundwater, lower degradation rates may result having exhausted the key electron acceptors or donors within a plume required for biodegradation to effectively proceed. The dominant access to such electron acceptors or donors might only then occur on the fringes of plumes, and indeed the longitudinal extent of plumes might be entirely controlled by dispersive mixing on the fringes of such plumes [41]. For example, for a petroleum groundwater plume made up of soluble components such as benzene, toluene, ethylbenzene, xylene (BTEX) and naphthalene, natural biodegradation was shown to be occurring under sulfate-reducing conditions, but that sulfate was strongly depleted in the core (inside) of the plume, while sulfate was abundant on the fringe and outside the groundwater plume [42]. Prommer et al. (1999) [43] quantified this for multiple electron acceptors and assessed the effect of seasonal flow direction changes on natural rates of biodegradation [44], confirming the strong dependence of biodegradation on the plume fringing processes, especially mixing limitations. The general shift in preferred electron acceptors during biodegradation of a petroleum hydrocarbon plume in groundwater and associated fringing reactions is shown in Fig. 2. In contrast, where all other electron acceptors are largely depleted methanogenesis may continue within the core of a plume.
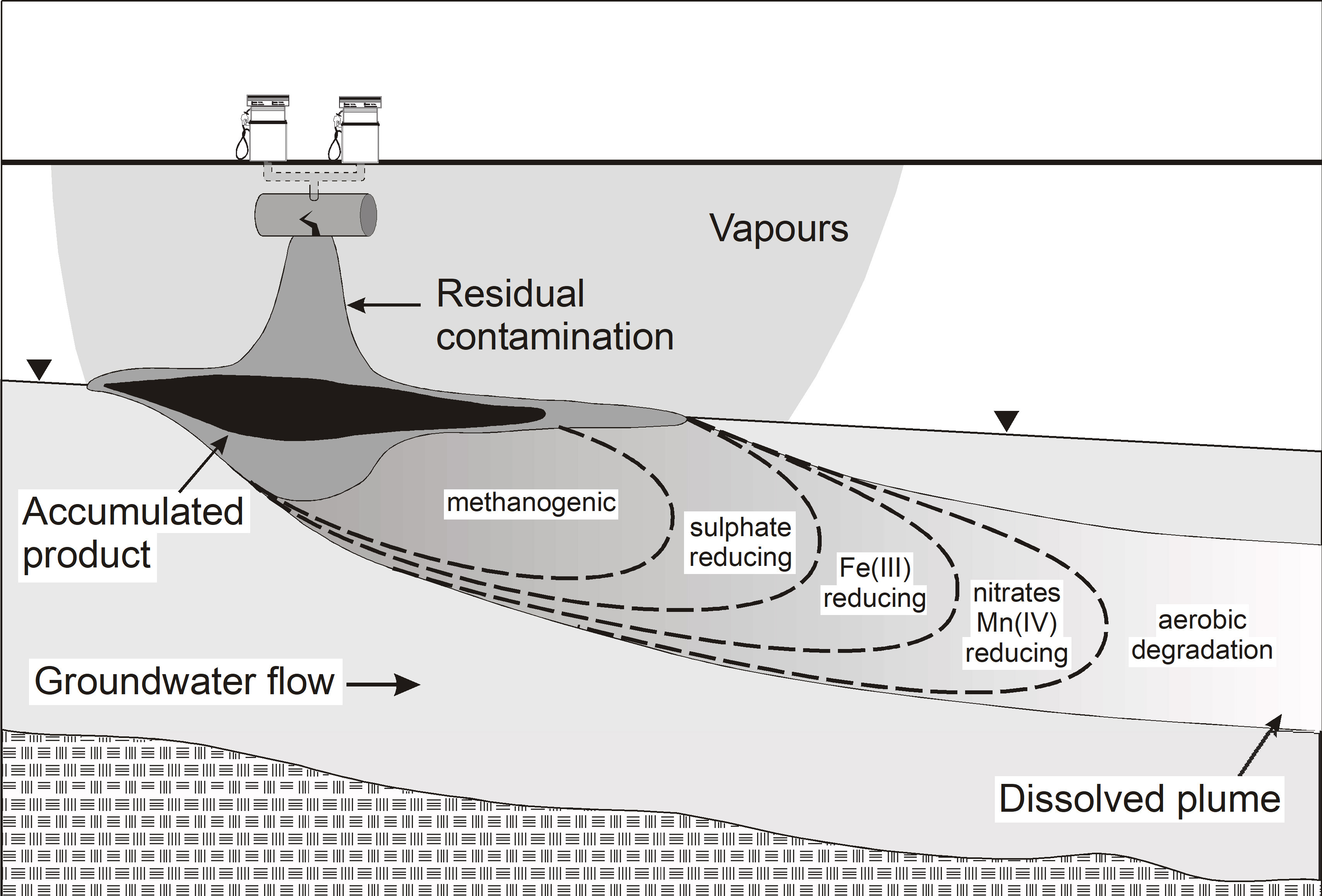
Schematic of groundwater zones of the possible sequential electron acceptor reactions during natural attenuation (biodegradation) of petroleum fuel compounds. Oxygen is consumed first by aerobic micro-organisms that biodegrade soluble components such as BTEX compounds. Once depleted the next electron acceptors (nitrate and Mn (IV)) start to be reduced by nitrate-reducing and manganese-reducing bacteria, and so on for the next electron acceptor, with methanogenesis common after depletion of the primary electron acceptors. Note that oxygen, nitrate and sulfate are dissolved electron acceptors, while Mn (IV) and Fe (III) occur as (solid) oxide precipitates on the aquifer media.
Indeed, Meckenstock et al. (2015) [45] argued for a stronger distinction between the concept of fringing processes and the longitudinal redox zonation of aquifers largely as depicted in Fig. 2. Redox zones may be less well defined or be absent where geochemical and microbial niches exist in aquifers, or where biodegradation is kinetically controlled allowing delays in consumption of electron acceptors/donors and hence allow overlap of redox zones. It may be that Mn (IV) or Fe (III) reduction may occur in overlapping zones with methanogenesis for example, although ‘energetically’ a cascading redox order would be dictated.
Initial studies to quantify biodegradation processes and rates involved recovery of groundwater and/or aquifer sediment samples to undertake laboratory-based batch microcosm or slurry investigations [46, 47, 48], and various scaled soil column or soil core investigations [24, 49, 50]. Such studies often added amendments (nutrients, electron acceptors/donors) to enhance biodegradation or to assess its potential. To measure degradation, either changes in the concentration of the parent contaminant was measured, or changes in one or more of the amendments that were added, and for some contaminants (e.g., tetrachloroethene (PCE)) degradation products such as vinyl chloride or even chloride as a final de-chlorination product were monitored [46, 51].
Such laboratory studies sometimes had limitations due to (i) experimental conditions not being able to preserve samples so as they continued to represent groundwater conditions (e.g., geochemistry, temperature); (ii) not being able to capture the finer and average scale of biodegradation across groundwater plumes, and (iii) for batch experiments, not representing groundwater flow conditions. The properties of the contaminant itself sometimes challenged accurate estimation of biodegradation mass losses due to mass losses onto experimental equipment (e.g., PFAS can sorb to glass vials) or during sampling (e.g., volatile organics like PCE can degas readily if a sample is opened to the atmosphere) [52]. Whilst necessary and informative of biodegradation processes and in discovering new underlying processes responsible for biodegradation, biodegradation rates estimated via batch laboratory investigations were cautiously taken as accurate representations of biodegradation rates within field groundwater plumes and have been viewed with caution when used in groundwater plume modelling [53].
To avoid laboratory limitations, investigations increasingly sought to measure in situ biodegradation processes and rates more directly in the field. Push-pull tests were developed, using boreholes to inject a prepared test solution into the subsurface and recover it measuring concentration changes over time during recovery of the injected solution [54, 55]. The time series of recovery showed depletion or enrichment of key tracers and contaminant concentrations that allowed estimation of biodegradation rates. Such tests have been used on a range of contaminant types and subsurface environments [56, 57]. Thierrin et al. (1995) [58] injected deuterated BTEX compounds into the center of a BTEX plume and tracked depletion of the deuterated compounds relative to a conservative tracer to assess concentration reductions and estimate biodegradation rates. This technique was repeated some years later for trichloroethene (TCE) and munition plumes in groundwater [59, 60]. In situ microcosm chambers were developed [61] and promoted [62] to estimate in situ biochemical rate measurements or for studying the activity of inoculated bacteria in a contaminated aquifer. The in situ microcosms were inserted to isolate and control exchange within a portion of a contaminated aquifer, in effect creating an in-field subsurface laboratory facility. Isotopic changes in parent compounds or degradation products have also been investigated at field sites to infer processes and rates more directly [63]. Techniques for investigation of microbial population changes have advanced significantly over 40 years, now with a range of genetic and molecular techniques and advanced bioinformatics to assess population types, distributions, and changes over time [64, 65]. Integration of measures and information across microbial communities, isotopic signals and other tracer and geochemical data is increasingly undertaken to understand degradation rates and biodegradation potential at sites [66].
Field investigations and measurements techniques have also advanced to better identify details of groundwater plume features and biodegradation processes. Monitoring and measurements progressed from sampling groundwater from long screened boreholes (3–30 m), which would provide average concentrations of geochemical indicators over such depth intervals, to short screened multi-depth groundwater samplers to enable the vertical definition of contaminants and key processes from within the core of plumes to across plume edges [42, 67, 68]. This has evolved more recently to more in situ measurement approaches and online probes for sensing and tracking groundwater plumes, with a recent review published [69]. The finer scale definition of groundwater plume dimensions has allowed greater insights and linkages between geochemical transitions, flow processes and bio-reactive processes – thus improving conceptualization of key processes for further exploitation for active bioremediation and management of contaminant plumes.
Here we provide a status update on knowledge of the biodegradation of a range of contaminants in groundwater, including organic compounds such as petroleum and chlorinated hydrocarbons, munition compounds, PFAS, and some pesticides, and inorganic compounds such as nutrients and metals.
For petroleum fuels and crude oil, natural biodegradation processes and rates have been investigated for decades [70, 71], and for plumes [29, 72] outcomes from groundwater investigations are now formalized in national guidelines in the USA, Australia, and the United Kingdom– often termed Monitored Natural Attenuation (MNA) [73, 74, 75]. MNA as depicted in Fig. 2 is inclusive of all attenuation mechanisms including dispersion (leading to lower concentrations), sorption (usually leading to delays in the transport of contaminant mass in groundwater), and biodegradation due to co-consumption of electron acceptors (leading to true mass loss or conversion within the groundwater). Challenges to implementation of MNA for petroleum hydrocarbons are (i) the need to demonstrate stable or shrinking plumes that are not crossing jurisdictional boundaries; (ii) that risks to human health and the environment are acceptable, e.g., from compounds such as benzene, and (iii) that data and information is of such a quality to enable quantitative MNA assessment.
Often MNA approval for management of a petroleum hydrocarbon plume also requires removal of the source of the plume, which is usually a non-aqueous phase liquid (NAPL) product held in the vadose zone or within the near-depth interval of the zone of water table fluctuation. Natural Source Zone Depletion (NSZD) is now often being used to manage LNAPL in the subsurface where NSZD rates are deemed high enough compared to active source removal techniques [76]. NSZD is revisited later in the paper when considering biodegradation in the context of holistic approaches to plume management.
Chlorinated organic compounds such as PCE, TCE, 1,2 dichloroethane (1,2 DCA) and others can be degraded to different degrees both aerobically and anaerobically. Outcomes of many early biodegradation studies are summarized in Wiedemeier et al. (1999) [29]. A strong focus has been reductive de-chlorination and the role of electron donors (such as natural organic carbon or carbon amendments such as toluene, acetate, hydrogen, emulsified vegetable oil [77, 78, 79]) in driving de-chlorination to ethene/ethane [80]. More specialized amendments such as poly-3-hydroxybutyrate have been evaluated to assess persistent release and supply of electron donors [81]. The organisms performing the de-chlorination are using the chlorinated compounds as electron acceptors to sustain growth [82]. Largely, the only microbes that have been found to fully degrade chlorinated ethene organics to ethene are members of the group Dehalococcoides [83].
A concern has been the sometimes lack of complete mineralization leading to the accumulation of the potentially more hazardous compound vinyl chloride (VC) as a degradation product. Studies have also shown that the less chlorinated hydrocarbons such as 1,2 DCA and VC do degrade under aerobic conditions both in laboratory and field studies [51, 84, 85]. Formalized national guidance in the United States integrates the information and approaches needed to assess the natural attenuation of chlorinated solvent groundwater plumes for regulatory compliance [86], but similar guidance does not seem available elsewhere. Whilst we have come to understand the drivers for degradation of chlorinated hydrocarbons in groundwater, the variability of redox conditions and electron acceptors vs electron donors needed to maintain biodegradation processes compared to petroleum compounds, provides greater uncertainty for regulators to sign off on MNA of chlorinated hydrocarbon impacted sites.
Pesticides and their precursors such as atrazine and chlorophenols [6], like chlorinated solvent compounds, are xenobiotic, being man made and largely introduced to the biosphere, compared say to petroleum compounds which are naturally sourced and somewhat ubiquitous. As such, microorganisms that come into contact with xenobiotic compounds may not initially have the capability to degrade them. For atrazine, such observations were made by Franzmann et al. (2000) [87] who identified that atrazine degrading microorganisms needed to be isolated and augmented into atrazine contaminated aquifer material to induce biodegradation of the atrazine. Under aerobic conditions atrazine was shown to be fully mineralized [50].
Other organic compounds commonly contaminating groundwater and found to biodegrade and transform are munition compounds, and for chemicals used in Defense applications such as perchlorate. For munition compounds, 2,4-dinitrotoluene (2,4-DNT) altered microbial communities during biodegradation [88], the potential for biodegradation of RDX (hexahydro-1,3,5-trinitro-1,3,5-triazine) was evaluated using a combination of differing redox incubation conditions [48]; and degradation of trinitrotoluene, 2,4-DNT, and 3-nitrotoluene was shown to occur in microcosms, with biodegradation occurring under nitrate-reducing conditions whilst creating intermediate metabolites under aerobic conditions [47]. For perchlorate (typically used in rocket fuel), prior research on chlorinated solvents enabled rapid advances in its understanding and treatment by targeting the use of electron donors to accelerate biodegradation rates [89]. An overview is given in Stroo and Ward (2009) [90].
A range of other emerging chemicals are also being actively investigated to determine their biodegradability in groundwater [91]. As mentioned, research indicates that some of the organic compounds that comprise the precursors to PFAAs will bio-transform though a recent review states “they cannot be degraded completely through a single biodegradation pathway” [92]. Also, biotransformation within PFAS plumes is a process that may not expedite remediation but may exacerbate remediation prospects by converting often lower mobility unregulated PFAS precursors to higher mobility, regulated PFAAs [93].
The nitrogen cycle is well known, and there is a long history of research on nutrient biotransformation in groundwater especially that of nitrogen species such as nitrate and ammonium [94]. Significant contamination of groundwater has occurred due to agricultural practices, landfills, urban sewage, industrial use and other sources [95]. Denitrification of nitrate (transformation of nitrate to nitrogen gas) commonly occurs where available carbon sources are present [96], although ammonification (conversion to ammonium) may sometimes occur. Denitrification may also occur in the presence of other reduced species such as sulfides [97]. Ammonium, in contrast, is stable under reduced geochemical conditions but under aerobic conditions will transform into nitrite and nitrate [96].
As with any contaminants (and metals in particular) general attenuation occurs due to dispersion but also by sorption to aquifer sediment surfaces or potential binding with mobile organic carbon. A range of metals can change their chemical status and behavior based on aquifer geochemical conditions [98]. Microbial reduction of metals such as chromium and uranium has been shown to produce both reduced soluble, mobile end products as well as insoluble non-motile end products [99, 100, 101]. Attenuation processes for a number of inorganic contaminants such as copper, lead, cadmium, and chromium in groundwater have been summarized [102]. Bioprocesses can alter pH and other geochemical conditions to induce sorption/desorption processes that might bind or release some metals, and bacterial sulfate-reduction can also lead to bioprecipitation of resultant insoluble metal sulfides onto aquifer sediments removing metals from groundwater plumes [103, 104].
The general features of contaminant biodegradability and biotransformation investigated over the last 40 years, and provided in overview in Section 4, provide a knowledge platform whereby we might alter groundwater geochemistry to enhance bioremediation processes, where natural rates of biodegradation may not be eliminating risks in an acceptable time frame. Based on understanding the likely geochemical and redox conditions controlling contaminant biodegradation, the challenge to inducing greater rates of degradation in groundwater to bioremediate plumes is to create or produce such conditions in the subsurface. Delivery of amendments to create such conditions is non-trivial. Here a number of bioremediation strategies and delivery methods are discussed.
Because of limited mixing and the laminar flow nature of groundwater systems liquid injection of amendments (be they electron acceptors/donors, nutrients, acidity, etc.) into aquifers to induce greater biodegradation rates may result in an isolated volume of the amendment in the aquifer due to hydraulic displacement of the targeted groundwater plume away from the well location(s) during the fluid injection phase. To overcome this and to enhance biodegradation and remediation, several strategies have been considered including (i) pulsed injection of liquid amendments to induce greater surface area contact zones between the injected volumes and the contaminant plume [105, 106]; (ii) recirculating groundwater pumping wells whereby wells (or screens within the one well) might extract and inject groundwater with added amendments prior to groundwater reinjection or to induce mixing [107, 108, 109]; (iii) establishment of a stationary or less mobile treatment zone in an aquifer across the groundwater plume flow direction that reacts with the contaminant of interest – such as permeable reactive barriers (PRBs) [79, 110, 111]; (iv) injection of reactive gases (such as oxygen, hydrogen) that might accelerate biodegradation but also might remove mass by other means [51, 112]; (v) bio-electrochemical systems which can stimulate the interaction of microbial metabolism with electrodes and can aid physical migration due to the electric field generated [113] and (vi) injection of slurry or placement of solid or particulate material to induce greater biodegradation [114, 115]. Advantages and disadvantages of some of these methods are collated in Table 1 (Ref. [106, 116]). In short, all methods are challenged by the often large scale of pollutant plumes in groundwater, because if plumes are greater than 100s of metres long and wide, close spacing of injection/extraction infrastructure or ongoing pulsing of injections might be prohibitively expensive. Rebound and back-diffusion effects can also prolong groundwater remediation timeframes. PRBs and other ‘across-plume’ injection or amendment placement strategies often enjoin fewer initial costs but only treat groundwater at selected cross-plume locations.
Bioremediation strategy | Advantages | Disadvantages |
Injection of liquid amendments (may be paired with extraction) | Largely uses above ground infrastructure that is easier to control; Potential treatment of larger aquifer areas depending on radius of influence of injection and when possibly paired with extraction wells. | May need to pulse to avoid displacing plume [106]; Ongoing operational and maintenance costs; Potential clogging of injection wells; Multiple injection locations likely needed if whole plume to be treated but may be distributed further using extraction wells; Where extraction is employed, surface disposal of residual pumped contaminants may be required. |
Recirculating wells | Largely uses above ground infrastructure that is easier to control; Potential treatment of larger aquifer areas depending on radius of influence and depths of injection/extraction in recirculation wells; Can target primary and secondary plume sources. | Ongoing operational and maintenance costs; Potential clogging of wells; Where pumped to surface, disposal of residual pumped contaminants; Multiple recirculation well locations likely needed if whole plume to be treated. |
PRBs | Treatment of all groundwater flowing past the installed location (suitable for site boundaries or compliance points); Potentially lower maintenance and operational costs. | Installation costs may be high if the depth of installation is large, if needed to be keyed into underlying strata and extending across the entire plume; Replenishment of the PRB bio-amendment may be required; Does not treat entire plume and treatment may be prolonged. |
Injection of gases | Only gas injection infrastructure needed; For oxygen, greater mass can be delivered in a gas phase compared to oxygen in liquid amendments and hence more cost effective; Gas injection might remove additional volatile mass beyond biodegradation; Could be configured as a PRB curtain. | Only suitable for contaminants where gases can induce biodegradation (e.g., oxygen, hydrogen); Multiple gas injection locations likely needed if whole plume to be treated to enhance bioremediation; Gas/air capture may be needed if volatilisation of contaminants is induced except if biosparging is successful [116]; Gas injection may reduce hydraulic conductivity and change groundwater flows. |
Injection/placement of slurry/slow release materials | Longer term release of bio-stimulants; Targeted emplacement; Potentially less cost if the reapplication is infrequent or replacement time is prolonged. | If placed in existing wells the zone of treatment is largely the diameter of the well (which would mean the installation of many wells); Lack of connectively of slurry injection may lead to plume bypass flow; Mostly oxygen and hydrogen release compounds are currently available; The effect on groundwater flows is uncertain. |
Despite the limitations mentioned in Table 1 across groundwater bioremediation strategies, below we provide a brief overview of two primary strategies and selected field case studies for PRBs and injection of gases, that highlight their potential, inclusive of some outcomes for bioaugmentation.
Bioaugmentation is an additional strategy for enhancing biodegradation [83]. Bioaugmentation is the addition of microbes into an aquifer to effect remediation and could be implemented as part of a number of the strategies in Table 1. The aim of bioaugmentation is to introduce specialized metabolic capabilities to induce biodegradation or transformation of specific compounds, or to bolster population sizes where they are limited and by adding other amendments alone may not increase the relative abundance of the desired biota. To be effective the augmented microbes must survive, grow, and retain their degradative capacity. Note that in many cases addition of microbes may not be needed since indigenous biota in groundwater are often capable of degrading contaminants if they have been resident for some time; geochemical and mass transport conditions usually limit biodegradation processes. One of the advantages of bioaugmentation is the ability to select the desired metabolic activity. This can be particularly beneficial where microbes might take considerable time to gain the capability to mineralize contaminants of interest (e.g., atrazine [87], and see the latter discussion). And, while bioaugmentation has become a more common treatment for sites contaminated with chlorinated solvents, it has not performed as well for other pollutants [83]. Bioaugmentation may be more successful in ex-situ systems such as bioreactors treating contaminated soil or groundwater. Disadvantages of bioaugmentation are that few successful field demonstrations have occurred beyond chlorinated solvents, it has been difficult (but becoming more feasible [117]) to monitor and differentiate introduced species from indigenous microbes, introduced species may be outcompeted by natural microbes upon addition to subsurface systems (in particular), microbes may lose their degradation capability upon injection, and transport and delivery of microbes to the target regions of an aquifer may be problematic. Regardless, via rapid progress in molecular biology capabilities and the genetic manipulation of microorganisms, new gene bioaugmentation holds promise [118].
Davis and Patterson (2003) [119] reviewed progress and developments with bio-treatment PRBs. They outlined operational needs and the potential for amendments into PRBs that could biodegrade and/or bio-transform petroleum hydrocarbon, ammonium/nitrate, chlorinated hydrocarbons, metals and other contaminants in groundwater. A number of studies have investigated the use of slow-release carbon sources in PRBs (e.g., sawdust [120, 121], cotton [122], peat [123], imitation vanilla [124], vegetable oil [79], hydrogen/methane [125], leaf mulch/wood chips [126], mulch [127]) for reductive bioremediation of contaminants such as nitrate, chlorinated solvents, munitions (e.g., RDX), sulfate (and low pH) and some metals in groundwater. Some PRBs have used oxygen release compounds placed across the pathway of BTEX plumes. Bianchi-Mosquera et al. (1994) [128] evaluated peroxide briquets to provide an electron acceptor for the biodegradation of injected benzene and toluene, Borden et al. (1997) [129] used immobilized nutrients and oxygen in briquet form; and Kao and Borden (1997) [130] used slow-release nitrate but observed that of the BTEX compounds benzene did not biodegrade. Apart from being able to identify the correct amendment that would create suitable conditions for enhanced biodegradation, linking groundwater flow through PRBs and ensuring adequate residence time for reaction kinetics is important for effective performance. Ensuring reduced hydraulic conductivities are not induced due to amendment emplacement within the PRB or through PRB clogging is also critical to not divert groundwater flows around the PRB treatment zone. To provide greater control on delivery rates and the spread of amendments in PRBs hollow fiber membranes and polymer tubes woven into mats have been evaluated in laboratory and field conditions [50, 96, 131].
The PRB scenarios depicted in Fig. 3 (Ref. [50, 96]) are for an atrazine plume [50] and an ammonium plume [96, 132]. For the atrazine PRB example (Fig. 3A), atrazine degrading microorganisms were isolated and bioaugmented into groundwater where atrazine biodegradation was not occurring [87], and to sustain the microbial community and stimulate bioremediation of the atrazine plume, oxygen was supplied by passing air through a network of polymer mat tubing providing an augmented and near constant oxygen supply [50]. A primary result is shown in Fig. 3C, indicating rapid biodegradation when oxygen was supplied via air injection through tubes woven into a polymer mat placed across the flow direction. The strategy was shown to be successful in achieving a short degradation rate half-life of about 0.35 days, compared to no biodegradation without oxygen amendment.
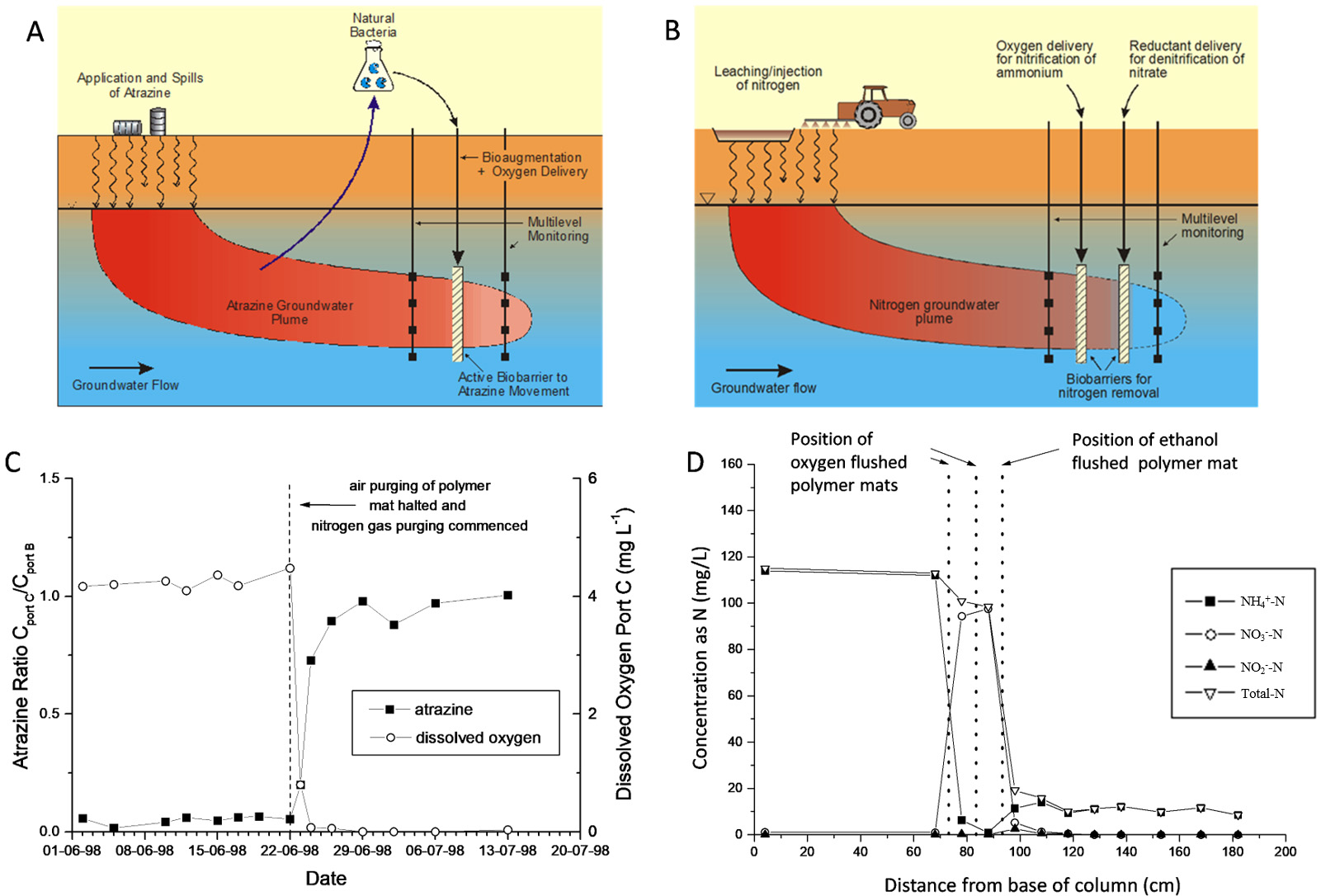
(A) Schematic of atrazine biaugmentation treatment barrier. In this scenario, an atrazine plume is being remediated by injection of atrazine-degrading bacteria and delivery of oxygen creating a permeable biobarrier wall at the leading edge of the plume. (B) Schematic of a dual (sequential) PRB to treat an ammonium plume - (i) to deliver oxygen to form nitrite/nitrate by nitrifying an ammonium plume, and subsequently (ii) to deliver a reductant (e.g., ethanol) to denitrify nitrite/nitrate to nitrogen gas. (C) Primary soil column result showing atrazine attenuation (decrease to near zero) immediately when oxygen was supplied in air at mid-way in the soil column (after [50]). (D) Primary soil column result showing ammonium being transformed during addition of oxygen and then nitrite/nitrate being degraded on addition of ethanol via the polymer mats (after [96]).
For the ammonium plume PRB example (Fig. 3B), a dual sequential treatment strategy was adopted to first induce nitrification of the ammonium plume by use of air addition through polymer mats followed by denitrification to nitrogen gas via addition of a reductant (e.g., carbon amendment or hydrogen gas) again via use of the polymer tubing mats. In Fig. 3D, primary data from the soil column investigations shows the successful sequential transformation with the second woven polymer tube mat supplying ethanol to denitrify the nitrite/nitrate generated earlier in the column. In laboratory columns, half-life nitrification rates (ammonium to nitrite/nitrate) were 0.07–0.25 days. Adding hydrogen sequentially to induce denitrification of the created nitrite/nitrate gave a half-life of 3.5 days, whilst using ethanol gave a half-life of 0.12–0.34 days [96]. The technique was implemented in the field in a funnel and gate PRB design using ethanol as the reductant [133].
A range of gas injection strategies have been adopted to remediate contaminants in the subsurface. Air sparging strategies (Fig. 4A, Ref. [112, 134]) were developed largely to remove volatiles such as BTEX or chlorinated volatiles but can also induce biodegradation. During air sparging of a dissolved gasoline plume, Johnstonet al. (1998) [112] found that aerobic biodegradation was occurring, but during the period of largest mass removal, biodegradation was occurring at a rate an order of magnitude less than volatilization rates estimated by capture of extracted volatiles. In the field study, air sparging started on day 10 but was stopped for 4-h periods on days 10, 11 and 13 to evaluate changes in dissolved oxygen and volatile organic compounds (VOCs) in groundwater. Data from the multi-depth monitoring location denoted DP2 (Fig. 4C), 1 m from the sparge well, show persistent reduction in benzene concentrations over time at shallower depths — say 3.5 and 4.5 m depths. Reductions in concentrations were observed deeper, but with significant rebound in concentrations when injection was halted. These depths appear to be towards the outer edge of the zone of influence for the sparge well in this sandy aquifer. Such data were used to both calculate biodegradation rates but also to optimize the spacing of sparge points across the gasoline groundwater plume at the site to effect remediation.
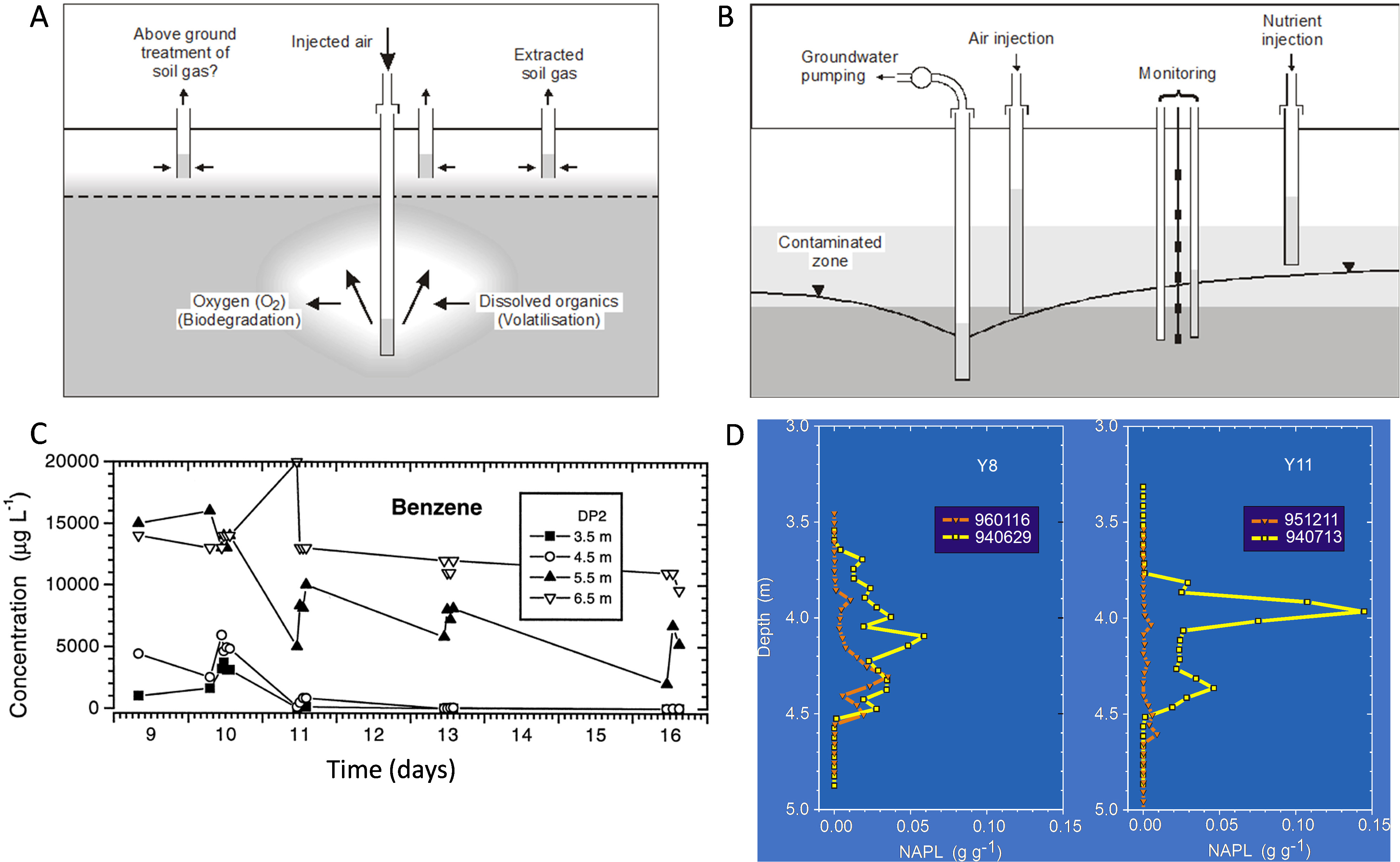
(A) Schematic of an air sparging system to remediate contaminated groundwater, showing the induced dual processes of volatilisation and biodegradation (where oxygen is utilized). (B) Schematic of bioventing remediation, showing air injection above the water table, drawdown of the water table to expose the zone of contamination to gaseous oxygen and nutrient addition through injection points. (C) Benzene concentrations during air sparging at multiple depths at monitoring well 1 m from the sparge well. Note that air sparging commenced on day 10 (after [112]). (D) Diesel NAPL in soil cores with depth below ground at two locations (Y8: where no nutrients were added; Y11: where nutrients were periodically added) from prior to 14 months of bioventing (yellow data), and at the end (orange data) (after [134]).
To maximize biodegradation and minimize the need to capture volatile emissions in extracted soil gas (Fig. 4A), biosparging strategies were developed using periodic gas sparging compared to continuous or high pressure/flows [116] avoiding the need to capture volatilized compounds. A similar approach was taken by Davis et al. (2009) [51] who tracked the production of chloride, bicarbonate and other indicators to quantify the aerobic biodegradation of 1,2 DCA and vinyl chloride in groundwater induced by air injection into a biosparging curtain across the groundwater flow direction. Other studies have investigated sparging/biosparging using reductant gases such as hydrogen to bioremediate chlorinated hydrocarbons [133, 135] and perchlorate and nitrate plumes [136]. An overview of air sparging and biosparging was published in Suthersan et al. (2017) [137].
Bioventing is the injection of gaseous amendments (usually air) above the water table (c.f., air sparging which is below the water table), often used to target contaminants like petroleum fuels residing at or across the zone of water table fluctuation (Fig. 4B) [138]. It can be well suited to removing significant mass from the source zone that might otherwise slowly dissolve and continue to be a source of soluble contaminants into a groundwater plume. Sometimes the water table is drawn down via pumping to expose the contaminated horizon to an injected gas phase, and nutrients might be added to increase biodegradation rates as depicted in Fig. 4B. Such a trial was implemented at a refinery site [139] for weathered diesel, and over 14 months biodegradation rates were calculated from oxygen consumption (amongst other measures) [134, 140]. Biodegradation rates increased over the trial from 5–15 mg/kg-soil/day up to 30–90 mg/kg-soil/day. Key outcomes are shown in Fig. 4D. Near complete diesel NAPL mass removal was achieved for the depth profile where nutrients (ammonium nitrate and some phosphorus) were added periodically (Y11). Drawdown of the water table and targeted nutrient delivery to the zone of contamination were seen as critical to successfully increasing biodegradation rates and mass removal at Y11. Significant mass removal also occurred where no nutrients were added (Y8) (Fig. 4D), but complete diesel mass removal over the time of bioventing did not occur at Y8.
Compilations of bioremediation performance data at field sites across all contaminants discussed here are not available, however assessments have been made for chlorinated solvent and petroleum hydrocarbons. Performance data across 117 chlorinated hydrocarbon anaerobic bioremediation projects showed a median reduction in source zone primary contaminant concentrations of 91% after the bioremediation project was implemented [141]. However, only 7% of the projects achieving the United States drinking water criteria. A similar compilation for petroleum hydrocarbon sites showed a median reduction in source concentration post bioremediation of just less than 90% with 11% of the projects achieving the U.S. drinking water criteria [142]. There are likely several caveats on the estimated means; one of which may be the dependence on mass removal effectiveness of prior site characterization efforts and the efficacy of the methods adopted to deliver amendments to contamination targets in the subsurface; another caveat may be that improved technologies, knowledge and approaches may have been available at more recent bioremediated sites compared to sites undergoing bioremediation initially. Never-the-less, improvements in bioremediation are needed to meet closure criteria in an efficient way and in targeting risk drivers.
In Section 2 we discussed features of groundwater systems and their role in biodegradation of a contaminant plume. The longevity and extent of groundwater plumes relies also on the ‘strength’ of the source of the plume [143] – i.e., both the contaminant concentration and the net flux of dissolved components at the source entering the plume. There are increasing efforts to quantify the source strengths of plumes, and to understand what mass depletion from source zones might be required to reduce concentrations below regulatory criteria [144], and fluxes to a level that would enable biodegradation and other attenuation mechanisms to accelerate the shrinkage of plumes. As mentioned earlier, for petroleum hydrocarbons, focus has moved from MNA of plumes to NSZD which seeks to quantify all groundwater and vadose zone biodegradation and mass loss including volatile losses [145, 146]. Longer term trends over time in NSZD whole-of-source biodegradation rates, and overall mass losses are being investigated [139]. Because of the low apparent biodegradability of PFAS many investigations are underway to quantify [19] and limit [147] the leaching of PFAS from source soils and vadose zones, to reduce the likelihood of exceeding low regulatory compliance criteria in groundwater and receiving water bodies. Indeed, even after primary plume sources are removed, secondary sources may lead to back-diffusion from tight, low-conductivity, or less mobile parts of aquifers and soils which can continue to feed groundwater plumes over long periods despite best efforts to remediate [148, 149]. Some remedial strategies have also targeted direct removal of source zone mass below the water table to seek to limit groundwater plume migration and length [150, 151] and to enable accelerated shrinkage of plumes via biodegradation processes [152]. Defining the level of effort required in remediating whole plumes to regulatory acceptance criteria requires quantitative integration of all key bio-geophysical and chemical processes within soil and plume source zones and throughout a groundwater plume’s extent (as per Figs. 1,2), and where needed inclusive of interactions and contact with receptors (e.g., ecosystems or groundwater extraction from wells). We provide an overview of some integrative modelling efforts in Section 6.3.
Models require input parameters such as the functional form of biodegradation rate kinetics. Biodegradation rates may change due to a range of factors, inclusive of the type and concentration of the parent compound being degraded, the growth, decay, and transition in suitable microbial populations [36, 153], the creation of degradation products, limitations due to nutrient or other carbon sources, competing contaminants when mixtures are present, temperature effects [154] or inhibitions due to toxicity of some contaminants. Early laboratory batch and microcosm studies simply graphed and analyzed concentration changes over time [155] to formalize kinetic functional forms [156]. A linear decreasing concentration over time implied a constant biodegradation rate unaffected over time and is termed zero-order kinetics [134, 140]. An exponentially decreasing concentration curve over time, was often fitted to a first-order kinetic rate meaning the rate was proportional to the concentration of the parent compound [157]. Second or nth order reaction kinetics have also been explored to fit observed data [158]. It is noted that concentration vs. time data from monitoring wells is not a biodegradation rate of dissolved constituents but more a reflection of source zone attenuation rates, and that concentration vs. distance rates reflect bulk attenuation coefficients that include primarily biodegradation with some dispersion [159].
Monod rate kinetics [160] are often used as they set a maximum asymptotic rate of reaction that reflects the metabolic capabilities of the microbial population. It is also a formulation that transitions between approximately first-order kinetics for low concentrations to zero-order rate kinetics for high concentrations. A number of studies have compared zero-order, first-order and Monod models in representing observed data [161, 162]. Chambon et al. (2013) [162] found when modelling reductive de-chlorination that zero-order and Monod kinetic parameters varied over a wide range, and that experimental microbial data were scarce. They surmised that the wide range of parameters was because reductive de-chlorination involved many microbial populations and reactions (e.g., fermentative, methanogenic, iron reducing) that were not accommodated by simple functional forms of the rate kinetics.
Monod kinetics are often used in groundwater biodegradation models and sometimes multiplications of many Monod kinetic functional forms are used to represent limitations and rate controls due to additional chemical species that may be needed in biodegradation reactions, or indeed those that might inhibit biodegradation [163, 164]. Rifai and Bedient (1990) [165] compared a dual substrate Monod kinetic relationship to calculate biodegradation to that of an instantaneous biodegradation rate where the rate is limited by transport processes (perhaps an electron acceptor or donor transported to the zone of contamination). They found that whilst the Monod model might be more accurate, it also required estimation of additional parameters. Borden et al. (1986) [166, 167] introduced the concept of instantaneous reaction kinetics for groundwater plumes whereby all oxygen and hydrocarbon would be largely instantly consumed by microbial controlled biodegradation only limited by the transport and availability of oxygen and hydrocarbons. This was similarly proposed for oxygen and hydrocarbon vapor biodegradation in vadose zones [168].
Kinetic rate formulations are simplified representations of biodegradation processes. Accommodating all the pathways and sub-processes responsible for contaminant biodegradation in kinetic models would lead to overly complex systems with a large number of unresolved parameters that would require estimation or fitting. Often the underlying detail of multiple microbial groups contributing to many sub-reaction pathways are unable to be accommodated [162]. Assumptions regarding kinetics and which key chemical species provide the appropriate geochemistry suitable for biodegradation to proceed are necessarily simplified in kinetic models.
The range of models that seek to simulate biodegradation processes in groundwater is vast [163, 166, 169]. Models that integrate groundwater flow aspects, geochemical reactions and adequately represents microbial biomass responses are necessary to constrain the potential natural rates of attenuation that may be estimated for aquifers, and indeed to evaluate designs for accelerating bioremediation. Simplified models have been developed (e.g., BIOSCREEN and BIOCHLOR [170, 171]) for approximating plume dimensions. These commonly assume groundwater flow rates and a degradation half-life. The dispersion coefficient is also a key parameter as a measure of dilution and mixing. However, such models can easily provide unrealistic estimates of biodegradation and hence plume length when key coupled processes are not incorporated and when not calibrated to actual site data.
Clement et al. (1998) [172] developed RT3D which described discrete transformation pathways for contaminants with known degradation pathways and applied it to a range of contaminants [173]. PHT3D [43, 174] was one of the first models to link multicomponent groundwater plume reactive transport modules to geochemical and redox controls on contaminant concentration changes during biodegradation. The framework also ensured limited numerical dispersion errors so as not to incorrectly overmagnify mixing and hence biodegradation within and on the fringes of plumes [44]. Linking the geochemical equilibration code PHREEQC provided additional indicators of biodegradation such as inorganic chemistry changes, but also assisted with constraining model simulations to more realistic site geochemical conditions [175, 176]. To additionally simulate microbial population mobility and attachment, Wang and Corapcioglu (2002) [177] modelled bioaugmentation using modified Monod kinetics and a microcolony concept to investigate the relative effects on the transport and biodegradation of an organic contaminant. Increasingly the dynamics of microbial populations and their role in biodegradation is being embedded into reactive transport models [178, 179]. New multi-omics tools promise vast data sets; accommodating it meaningfully in reactive transport models to advance the understanding of biodegradation processes is a future challenge.
Comparison of model outputs to laboratory or field data is key to determining if models accommodate most of the underlying processes being investigated. When fitted adequately to field or laboratory data [164, 180, 181, 182, 183, 184, 185] the model provides a diagnostic tool to assess scenarios, to predict future trends and contaminant plume fate — but also provides a platform for assessing potential remedial options and designs to accelerate and optimize bioremediation of groundwater plumes [186, 187, 188]. When not calibrated or constrained by data, model predictions can be uncertain, and use of such models may pose a risk in assessing underlying processes incorrectly. Appropriately calibrated models are not only becoming more powerful and representative of biodegradation processes in groundwater — but are essential to addressing the challenges of managing the risk and remediation of contaminant plumes in groundwater, and groundwater quality more generally [183].
Understanding of the biodegradation of polluting chemicals in groundwater and associated microbial community capabilities has advanced significantly over 40 plus years. It has been shown that microorganisms in aquifers can constrain and, in many cases, remove contaminant plumes from groundwater, being particularly successful for petroleum hydrocarbons [189], somewhat so for chlorinated hydrocarbons and nutrients, and been shown to transform and remove some metals from groundwater. Research on PFAS is only recent, with evidence of biotransformation for PFAS precursors to PFAAs, but PFAAs have not been shown to degrade or otherwise transform under ambient environmental conditions. Engineered bioremediation strategies can be highly effective at degrading significant contaminant mass and lowering concentrations of groundwater plumes, although collated performance across sites also shows that meeting regulatory criteria across all risk drivers remains problematic.
Key lessons learnt include:
GBD designed the research study, analyzed the information, and wrote and edited the manuscript. GBD contributed to editorial changes in the manuscript. GBD read and approved the final manuscript. GBD has participated sufficiently in the work and agreed to be accountable for all aspects of the work.
Not applicable.
Colleagues at CSIRO and within industry and government agencies are thanked for their support over many years to undertake important laboratory and field investigations related to biodegradation of contaminants in groundwater. Colin Johnston, Peter Franzmann, Bradley Patterson, Henning Prommer and John Rayner have contributed significantly to laboratory, field and modelling efforts to progress insights. Four reviewers of a previous draft of the paper are also acknowledged.
This research received no external funding.
The author declares no conflict of interest.
Publisher’s Note: IMR Press stays neutral with regard to jurisdictional claims in published maps and institutional affiliations.