- Academic Editor
Background: Different types of stress inflicted in early stages of life elevate the risk, among adult animals and humans, to develop disturbed emotional-associated behaviors, such as hyperphagia or depression. Early-life stressed (ELS) adults present hyperactivity of the hypothalamus-pituitary-adrenal (HPA) axis, which is a risk factor associated with mood disorders. However, the prevalence of hyperphagia (17%) and depression (50%) is variable among adults that experienced ELS, suggesting that the nature, intensity, and chronicity of the stress determines the specific behavioral alteration that those individuals develop. Methods: We analyzed corticosterone serum levels, Crh, GR, Crhr1 genes expression in the hypothalamic paraventricular nucleus, amygdala, and hippocampus due to their regulatory role on HPA axis in adult rats that experienced maternal separation (MS) or limited nesting material (LNM) stress; as well as the serotonergic system activity in the same regions given its association with the corticotropin-releasing hormone (CRH) pathway functioning and with the hyperphagia and depression development. Results: Alterations in dams’ maternal care provoked an unresponsive or hyper-responsive HPA axis function to an acute stress in MS and LNM adults, respectively. The differential changes in amygdala and hippocampal CRH system seemed compensating alterations to the hypothalamic desensitized glucocorticoids receptor (GR) in MS or hypersensitive in LNM. However, both adult animals developed hyperphagia and depression-like behavior when subjected to the forced-swimming test, which helps to understand that both hypo and hypercortisolemic patients present those disorders. Conclusion: Different ELS types induce neuroendocrine, brain CRH and 5-hydroxytriptamine (5-HT) systems’ alterations that may interact converging to develop similar maladaptive behaviors.
Different psychiatric disorders such as depression, anxiety and hyperphagia-induced obesity [1, 2, 3, 4, 5] develop during adulthood due to alterations in the hypothalamic-pituitary-adrenal (HPA) axis function [6, 7, 8, 9] resulting after being exposed to a variety of stressful events during early life periods.
Threatening experiences in early childhood, such as abandonment, neglect, physical or sexual abuse, parental illnesses or poverty, among other adversities, induce the disruption of the HPA axis functioning, given that it is a particularly susceptible period to be impaired by stressful events [8].
Chronic exposure to early-life stress (ELS) is specifically associated with hyperactivity of the HPA axis characterized by elevated glucocorticoids serum levels (cortisol in humans, corticosterone (CORT) in rodents), along with an impaired negative feedback regulation of the axis, recognized by high hypothalamic corticotropin-releasing hormone (CRH) mRNA and protein content in the paraventricular nucleus of the hypothalamus (PVN), which contains the CRH neurons involved in directing the functioning of the neuroendocrine axis [10]. The high serum glucocorticoids levels in early life periods, when sustained during long terms, are also able to disrupt different brain neuropeptides and neurotransmitters’ systems, such as those of CRH and serotonin (5-hydroxytriptamine, 5-HT) particularly in the amygdala (Amy) and hippocampus (Hipp) [11, 12].
Amygdalar and hippocampal CRHergic neurons project their axons to the hypothalamic PVN and are known to activate and inhibit, respectively, the functioning of the HPA axis. Thus, alterations in the expression of their components, such as that of Crh, type 1 corticotropin-releasing hormone receptor (Crhr1), and glucocorticoids receptor (GR) of those regions, may account for the impairments found in neuroendocrine and neurobiological systems in early-life stressed offspring that persist until adulthood [3, 9, 13, 14] and that underly the development of depression or hyperphagia-induced obesity [4, 15].
Besides that of CRH, alterations in the serotonin system are also involved in the development of depressive-like behaviors and in stress-induced hyperphagia [11, 12]. In fact, CRH itself, as well as corticosterone, are able to modify 5-HT neurotransmission, supporting the assumption that early-life stress-induced impairments in CRH and in 5-HT systems are linked to specific maladaptive behavioral outcomes in adults.
However, although the participation of altered amygdalar, hippocampal and hypothalamic CRH and 5-HT pathways in the development of psychiatric disorders is well accepted, there is no description of specific changes to their components that may account for various behavioral maladaptations that lead to depression or to hyperphagia-induced obesity among adults that suffered from early-life stress events.
Therefore, we subjected rats to two different models of early life stress that mimic childhood abandonment (maternal separation, MS) or neglect (limited nesting material, LNM) and evaluated their food intake and body weight since weaning and up to adulthood, as well as their depression-like behavior in adulthood by subjecting them to the forced swim test (FST). FST is a paradigm to evaluate neurobiological changes related to coping strategies for stressors [16]. It is reported that if a stressor persists, it may increase the vulnerability to develop depression-like behaviors [17]. From this point of view, forced swim could be adequate to detect behavioral changes induced by early-stress interventions, such as the increase of immobility behavior [18]. MS consists in separating the offspring from their mothers 3 h daily during post-natal days 2 to 14 [19], whereas LNM refers to an erratic behavior of dams resulting from having limited amount of nest material during lactation [20]. Those paradigms induce fragmented maternal care in the weaning dams that increases their corticosterone serum levels and those of the offspring, leading to HPA axis alterations known to underly depression-like behavior, as well as increased food intake and body weight, in ten-week old adult rats [15]. Groups with or with no performance on the FST were sacrificed at the same time, and their trunk blood collected for evaluating corticosterone serum levels as evidence of stress and of the response to an acute challenge, the FST. Also, we extracted the brains and analyzed the genes’ expression of Crh, Crhr1, GR, as well as the activity of 5-HT pathway in PVN, amygdala, and hippocampus of adult rats, and compared those parameters between groups from the two different early-life stress paradigms and between animals with or with no performance of the FST.
We hypothesized that both groups of rats will show differential behavioral alterations linked to specific modifications in HPA axis-functioning parameters, as well as in the expression of brain CRHergic system components or in serotonin pathway activity that explain the diversity of mood disorders developed in adults exposed to various early-life adversities.
Twelve pregnant Wistar rats in the third week of gestation were obtained from
the animal house of the Instituto Nacional de Psiquiatría Ramón de la
Fuente Muñiz (INPRFM), and maintained in a 12 h light-dark cycle (lights on
07:00–19:00 h) under controlled temperature (22
(1) Control group (C): Dams and pups (n = 4) were left undisturbed and, after weaning (PND 21), dams were euthanized by decapitation, and pups were individually housed and left undisturbed until PND 71.
(2) Maternal separation group (MS): From PND 2 to 14, dams (n = 4) were withdrawn from their home cages to an adjacent room, while pups were left in their home cages for 180 min/day during the light period (10:00 to 13:00 h). At the end of the separation period, dams were returned to the nest cage. On PND 15, dams and pups were left undisturbed and housed in cages like C group. After weaning (PND 21), dams were euthanized, and pups were individually housed and left undisturbed until PND 71.
(3) Limited nesting material group (LNM): From PND 2 to PND 9, dams (n = 4) and their pups were placed on a bored metal surface 2.5 cm above the cage floor that contained sawdust to pick up droppings throughout the day. The only material offered to the dams to build the nest was one paper towel, according to the model described by Baram’s group [21]. On PND 10, the metal surface was removed, and dams and pups were housed in cages like C group. After weaning (PND 21), dams were decapitated, and pups were individually housed and left undisturbed until PND 71.
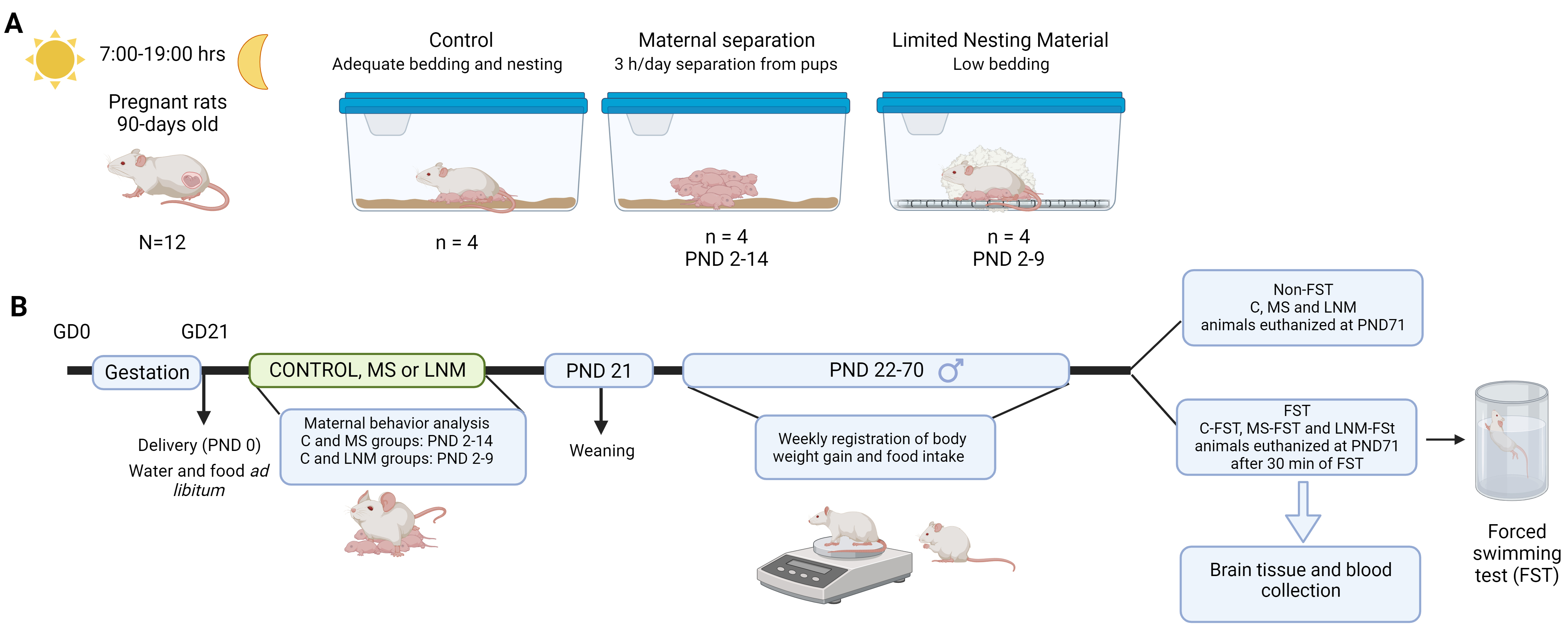
Experimental design. (A) Housing conditions of lactating dams and offspring. Pregnant rats were checked for delivery that was considered postnatal day (PND) 0. After delivery, dams and offspring were divided in control group (C: adequate bedding material and all the time together in the same cage), maternal separation group (MS: dams were withdrawn from home cages 3 h/day from PND 2 to 14), and limited nesting material group (LNM: from PND 2 to 9, dams and pups were together but the material provided for build the nest was not enough). (B) Timeline of the experimental design. After delivery (PND 0), rats were subjected to C, MS or LNM conditions; after weaning, dams were euthanized and pups were singly housed and left undisturbed until PND 71 when a subset of C, MS and LNM rats were euthanized, and another subset of rats were subjected to the forced swimming test (FST; C-FST, MS-FST, LNM-FST), and after 30 min of FST, they were euthanized. Brains and blood were collected. Created with Biorender. GD, gestation day; MS, maternal separation; PND, postnatal day; LNM, limited nesting material; FST, forced swim test.
At PND 21, all dams were sacrificed to measure corticosterone serum levels and adrenal glands’ weight. Male offspring (n = 12/group) were weaned, single housed, and maintained under controlled conditions with ad libitum access to food and water up to adulthood (PND 70). Since single housing is considered social isolation and a stressful condition, all our animals in fact were exposed to two different stressors at different periods of life [22]. Offspring food intake and body weight were registered on a weekly basis. On PND 71, a set of animals from each group (4 rats/group) were euthanized by decapitation (C, MS, LNM) and another set of rats (8 animals/group) were subjected to the FST and euthanized after 30 min (C-FST, MS-FST, LNM-FST). Trunk blood was collected, and the serum was obtained by centrifugation at 3000 rpm and used to analyze corticosterone levels. Brains were excised and stored at –70 °C until analyzed for Crh, Crhr1 and GR mRNA expression (n = 4/group), or for 5-HT and 5-hydroxyindole acetic acid (5-HIAA) content (n = 4/group). PVN, Amy and Hipp were hand dissected from coronal slices of frozen brain: for PVN and Amy –1.08 to –3.24 mm from bregma, for Hipp –3.72 to –5.16 mm from bregma [23]. The right hemisphere was used for mRNA content determinations, and the left hemisphere was used for 5-HT and 5-HIAA measurements.
Maternal care behaviors were analyzed in C, MS, and LNM dams. All animals were videotaped for a 30-minute period between 8 AM and 2 PM and different behaviors were analyzed. For MS group, the recordings were made after returning dams to home cages and compared to a control dams’ group who were separated from their pups for 5 minutes from PND 2 to 14. For LNM rats, we recorded the animals’ behavior in their home cages from PND 2 to 9. We assessed the spent time of mothers engaged in different behaviors, such as being near the litter, outside the nest, grooming the pups and themselves, passive nursing, and arched-nursing posture. We also counted the number of pups that the dams left out of the care area while nursing [24].
On PND 70, a subset of animals (n = 8/group) were allowed to swim for 15 min
(pre-test) in a modified version of the Porsolt’s FST (C-FST, MS-FST, LNM-FST
groups) [25]. Briefly, each rat was placed in a glass cylinder (46 cm tall
We used 50 µL of each rat’ serum to determine corticosterone content using a radioimmunoassay (RIA) kit (Coat-a-Count, Siemens, Los Angeles, CA, USA): limit of detection 5.7 ng/mL, inter and intra-assay variation 15 and 13%, respectively.
For total RNA extraction, frozen PVN, Amy and Hipp were homogenized in 4 M
guanidine thiocyanate (ICN Biomedical Research Products, Aurora, OH, USA) and treated as described [28]. RNA
quality was confirmed by 260/280 nm and 260/230 nm ratios of O.D. absorbance, in
addition to an agarose gel electrophoresis to analyze RNA integrity by
quantifying 28S/18S ratio, considering an optimal extraction when both values
were
Gene name | Protein | Sense primer (5′-3′) | Antisense primer (5′-3′) | Product length (bp) | NCBI reference sequence |
Nr3c1 | Glucocorticoids receptor | AAAAAGCACATCACACATAAATCTG | TAAATAAGAGGGAGCAAACTACTGG | 688 | NM_012576.3 |
Crh | Corticotropin releasing hormone | AGAAGAGAGCGCCCCTAAAC | ATCAGAATCGGCTGAGGTTG | 190 | NM_031019.2 |
Crhr1 | Corticotropin releasing hormone receptor 1 | TCCACTACATCTGAGACCATTCAGTACA | TCCTGCCACCGGCGCCACCTCTTCCGGA | 248 | NM_030999.5 |
Ppia | Cyclophilin | GGGGAGAAAGGATTTGGCTA | ACATGCTTGCCATCCAGCC | 257 | NM_017101.1 |
RT-PCR, reverse transcriptase polymerase chain reaction; NCBI, National Center for Biotechnology Information.
The number of cycles was optimized for each primer in a particular region. In the Amy, 29 cycles were used for pro-CRH, 31 cycles for CRH-R1, 30 cycles for GR, and 21 cycles for CYC. In the Hipp, 30 cycles were performed for pro-CRH, 32 cycles for CRH-R1, 29 cycles for GR, and 21 cycles for CYC. In the PVN, 28 cycles were used for pro-CRH, 30 cycles for CRH-R1, 27 cycles for GR, and 21 cycles for CYC. Each cycle consisted of 1 min at 94 °C, followed by 1 min at 64 °C for GR or CYC, 55 °C for CRH-R1 or 63 °C for pro-CRH, and a final minute at 72 °C. All cDNAs had a final extension of 10 min at 72 °C. PCR products (10 µL of each problem gene, and 5 µL of CYC) were separated by 2% agarose gel electrophoresis (Ultra-pure Bio-Rad, Hercules, CA, USA), stained with ethidium bromide (1 mg/L; Sigma-Aldrich, St. Louis, MO, USA), and revealed under a UV light. The optical density (O.D.) was measured with the Advanced American Biotech Imaging software (American-Applied Biotechnology, Fullerton, CA, USA), and the relative expression of mRNA was calculated as the ratio of each OD problem gene over that of CYC.
C, MS, and LNM adult offspring samples were homogenized in ice-cold 0.1 M perchloric acid (Sigma-Aldrich, St. Louis, MO, USA) (PVN in 400 µL, Amy in 500 µL and Hipp in 600 µL), and a 30 µL aliquot was used to determine protein content by the Folin-Ciocalteu method [30]. The remaining homogenate was filtered to dispose of impurities (0.25 µm pore, Whatman, Piscataway, NJ, USA).
High-performance liquid chromatography (HPLC) system consisted of an
auto-sampler (Waters Alliance 2695, Milford, MA, USA) equipped with a
Phenomenex® pre-column to filter impurities, an analytical
Phenomenex C-18 column (150
Standard solutions were prepared from a 1 mg/mL stock standard of each analyte and dissolved in ice-cold 0.1 M perchloric acid. Retention time was 7.2 minutes for 5-HT standards and 2.9 min for 5-HIAA standards. Data was acquired using the Waters Empower software for HPLC (Milford, MA, USA).
Differences between groups of dams in corticosterone serum content and pups’
caring behavior were analyzed by one-way analysis of variance (ANOVA).
Comparisons of body weight and food intake from weeks 3 to 10 after weaning were
analyzed by two-way repeated measures ANOVA. The scores of behavioral parameters
(FST) were analyzed by one-way ANOVA, while differences between pups’ groups in
adulthood for gene expression, serotonin metabolism, and corticosterone serum
levels were analyzed by two-way ANOVA (groups with no FST and those subjected to
FST). When p
Serum CORT levels of dams subjected to MS and LNM were elevated to 330 and
223%, respectively, compared to C dams (100%; 178.9
Group | ||||
Control | MS | LNM | ||
Cort (ng/mL) | 178.9 |
591 |
399.6 | |
Adrenal glands (g/kg b.w.) | 0.3 |
0.31 |
0.31 | |
Maternal care (min) | ||||
Outside the nest | 12.6 |
5.9 |
12.3 | |
Passive nursing | 6.3 |
11.6 |
9.8 | |
Arched nursing posture | 1.2 |
0.9 |
2.3 | |
Grooming the pups | 7.4 |
11 |
4.0 | |
Self-grooming | 2.7 |
0.7 |
1.6 | |
Pups outside the nest | 1.3 |
1.2 |
3.3 | |
Total number of male pups PND 1 | 16 | 16 | 12 | |
Total number of female pups PND 1 | 15 | 16 | 12 |
Data are presented as mean
Accordingly, the weight of adrenal glands of the same dams increased to 125 and
128% in MS and LNM dams, respectively, compared to that of C dams (100%; 0.25
We analyzed the stress effect on maternal behaviors. We found that the time MS
dams spent outside the nest was 47% less than C (100%; 12.6
Both MS and LNM offspring displayed more body weight than C group, reaching 12
and 24% at adulthood (week 10; C = 100%, 202
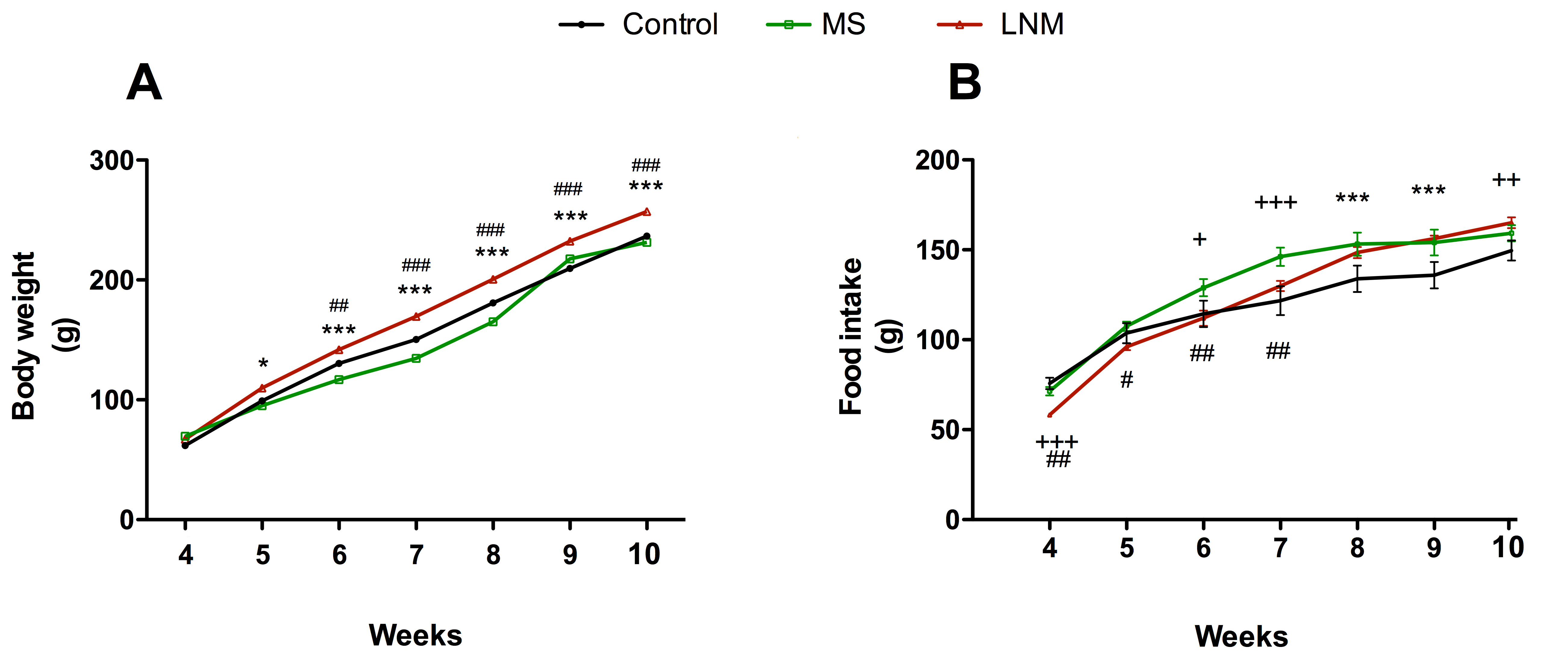
Body weight and food intake of control (C), maternal separation
(MS), and limited-nest material (LNM) offspring from weeks 4 to 10 after
delivery. (A) Body weight from 3 to 10 weeks. (B) Weekly food intake from 4 to
10 weeks. Data are expressed in grams (g) and presented as the mean
Regarding food intake, MS rats ingested 13% more food than C group only between
weeks 6 and 9 (C= 100%; week 6: 114
Swimming and climbing behaviors were not different between groups subjected to
FST (Table 3). However, both MS-FST and LNM-FST rats exhibited a 54% higher
immobility behavior when compared to C rats (100%, 13
Frequency | Group | ||
Control | MS | LNM | |
Immobility | 13.0 |
20.1 |
20.2 |
Swimming | 34.9 |
30.3 |
30 |
Climbing | 12.1 |
9.5 |
10.7 |
Frequency is referred as the total 5 sec counts of each behavior in the FST over
a 5 min period. n = 8 rats/group. Data represent the mean
The MS group showed an increase of 197% in CORT serum content when compared to
those of C animals (100%; 236
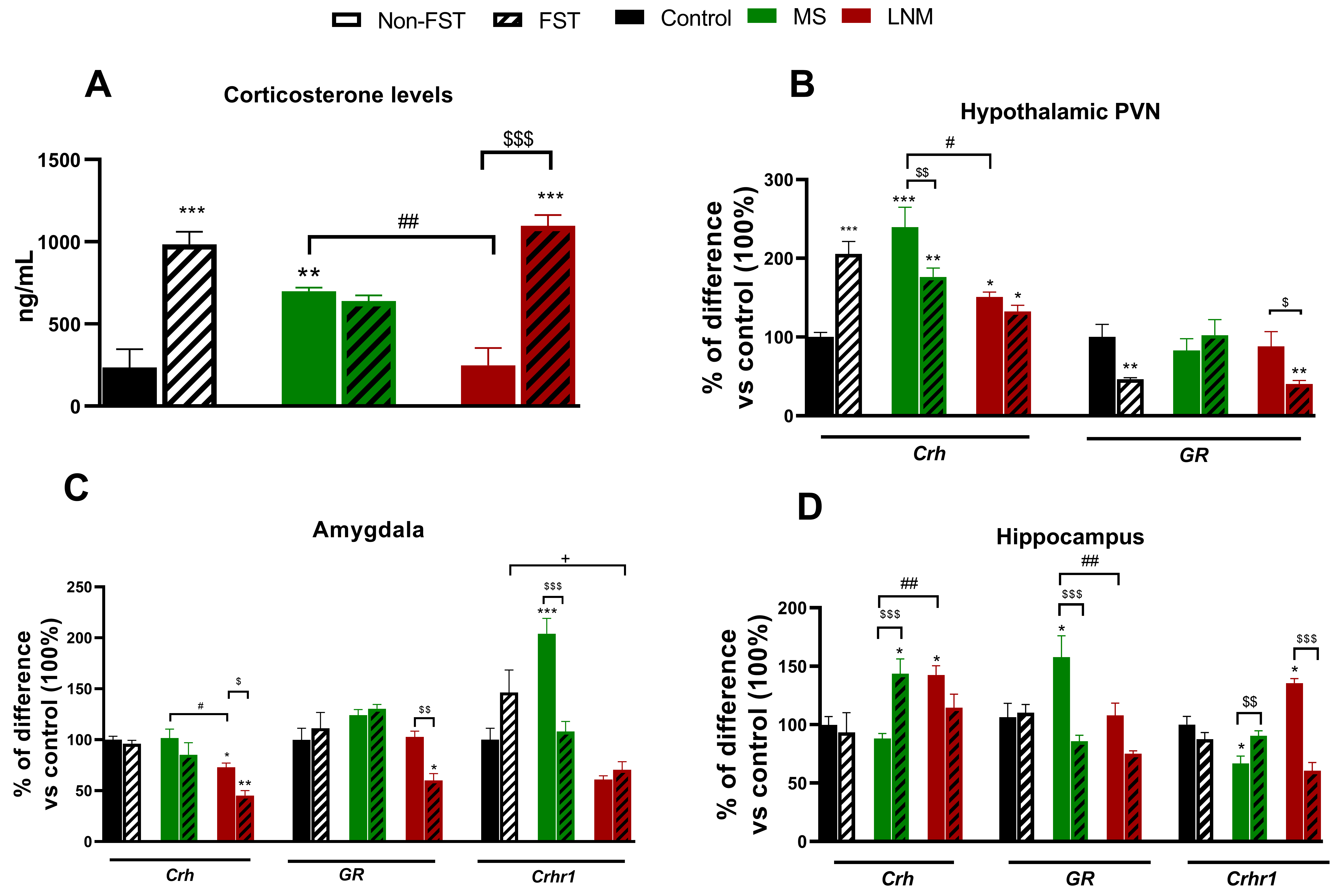
Corticosterone serum content and central gene expression of
Crh, GR and Crhr1 in control (C), maternal separation (MS), and limited
nest material (LNM) adult offspring that were or not subjected to the forced
swimming test (FST). (A) Serum corticosterone levels (ng/mL); mRNA expression of
Crh, GR and Crhr1 expressed in percentage of difference vs C in (B)
hypothalamic paraventricular nucleus (PVN), (C) amygdala and (D) hippocampus of
non-FST rats (C, MS and LNM) (open bars) and rats subjected to the forced
swimming test (FST; C-FST, MS-FST, LNM-FST) (lines in bars), n = 4–6 rats/group.
Data represent mean
MS and LNM groups increased Crh mRNA content in the PVN to 241 and
150% respectively, compared to that of C animals (100%, 1.3
Regarding GR mRNA levels, MS and LNM rats that were not subjected to
the FST had not changed. In contrast, C-FST and LNM-FST decreased GR
expression in the PVN to 46% and 40% to that of C group (100%, 3.13
In the Amy of LNM rats, we found a reduction of 27% in Crh mRNA
expression compared to C group (100%; 1.07
In the Hipp, we observed that LNM rats increased Crh expression to
142% when compared to that of C group (100%; 0.87
The levels of 5-HT in the PVN of rats exposed to LNM were 345% higher than
those of the control group (C = 100%; 4.73
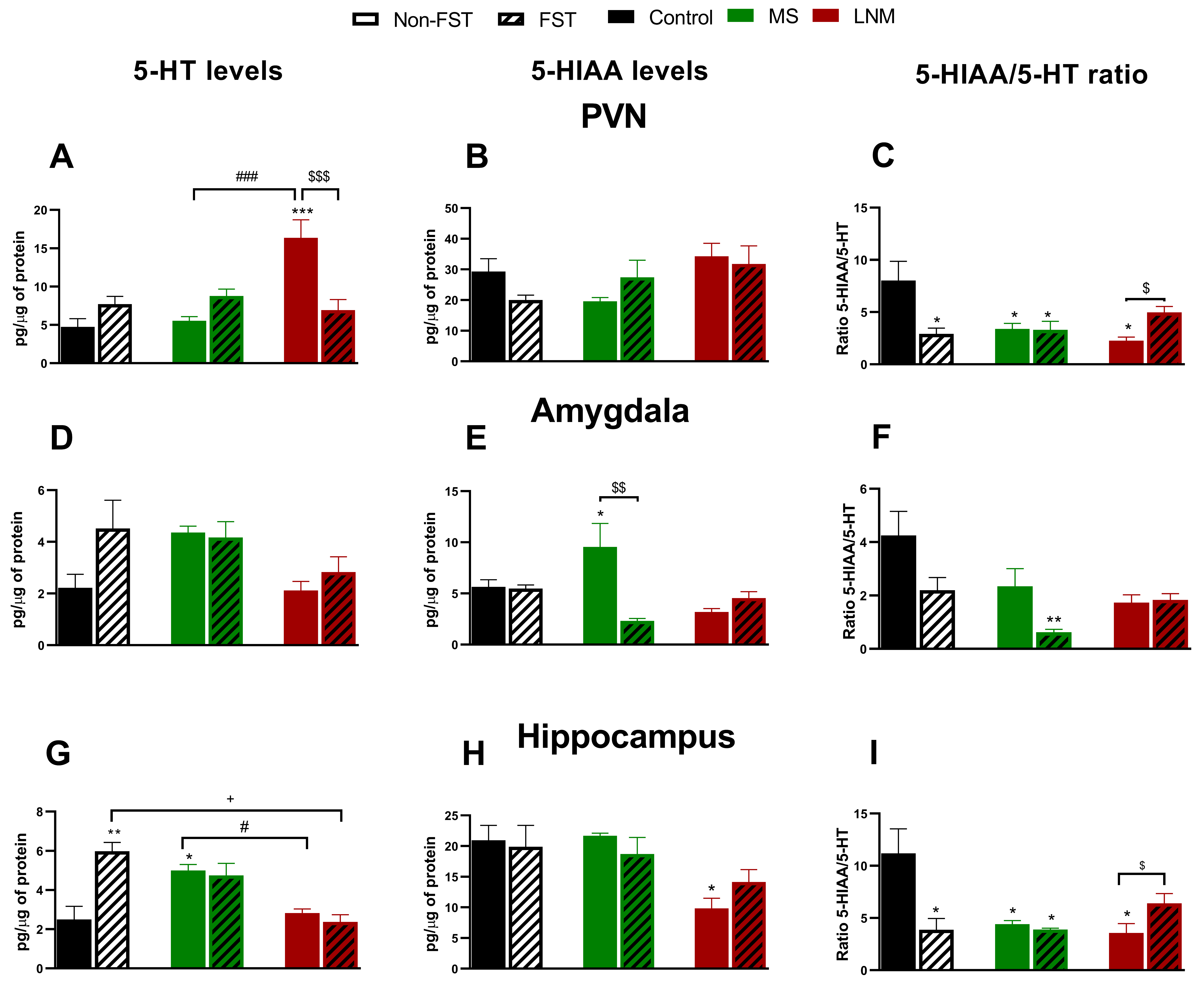
Central serotonergic system in control (C), maternal
separation (MS), and limited nest material (LNM) adult offspring that were or not
subjected to the forced swimming test (FST). Serotonin (5-HT) and
5-hydroxyindoleindole acetic acid (5-HIAA) levels
(pg/µg protein), and 5-HT/5-HIAA ratio in (A,B,C) hypothalamic
paraventricular nucleus (PVN), (D,E,F) amygdala and (G,H,I) hippocampus of non-FST rats
(C, MS and LNM) (open bars) and rats subjected to the forced swimming test (FST;
C-FST, MS-FST, LNM-FST) (lines in bars). n = 4 rats/group. Data represent the
mean
In the Amy, there were no changes in 5-HT content (Fig. 4D). In contrast, the
metabolite of serotonin 5-HIAA increased to 150% in MS group when compared to C
rats (100%, 6.37
Hippocampal 5-HT levels increased to 200% in MS group vs C animals (100%; 2.49
Although different early-life stress paradigms induced different HPA axis maladaptations (Fig. 5), the behavioral outcomes are similar, which show that mental illness, such as depression, may arise from different stressful events suffered during the early postnatal period.
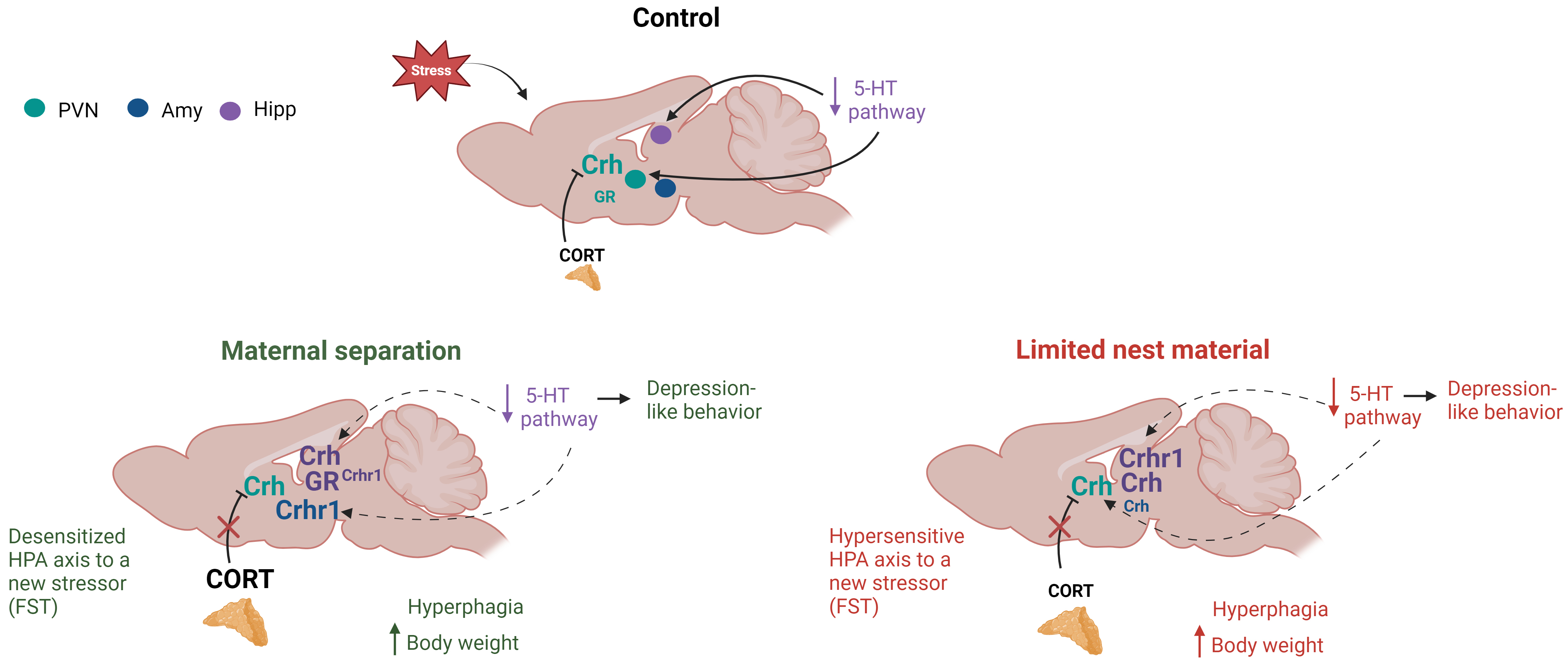
Summary of the effects of two different early-life stress (ELS) paradigms on the hypothalamic-pituitary-adrenal (HPA) axis and serotonergic pathway activity. The image shows a sagittal section of a rat brain, highlighting three cerebral regions: the hypothalamic paraventricular nucleus (PVN), amygdala (Amy), and hippocampus (Hipp). When control animals were subjected to an acute stressor (forced swimming test, FST), the HPA axis was activated, resulting in higher levels of CRH and lower levels of serotonin in the PVN. Maternal separation induced a desensitization of the HPA axis to a new stressor (FST), which caused depression-like behavior, hyperphagia, and an increase in body weight associated with a low serotoninergic activation. On the other hand, limited nesting material promoted a hypersensitized HPA axis to a new stressor (FST), leading to hyperphagia, increased body weight, depression-like behavior, and a decrease in the serotonin pathway. Dashed lines depict a decrease in serotonergic pathway, and bigger letters show an enhanced synthesis or content of CRH system’s components or corticosterone (CORT) serum content, while smaller letters represent a decrease in those parameters. Corticotropin releasing hormone (Crh), glucocorticoid receptor (GR), corticotropin releasing hormone receptor 1 (Crhr1), corticosterone (CORT), serotonin (5-HT).
The elevated CORT serum levels observed in dams from MS and LNM models positively correlated with their enlarged adrenal glands, supporting that they were chronically stressed during the weaning period. As a consequence, they showed alterations in their maternal care, which were different between MS and LNM dams: MS dams spent less time on the nest, but seemed to compensate it with higher offspring care, whereas LNM dams showed a more erratic behavior, since they left a greater number of their offspring outside of the caring place and groomed them more sparingly than MS dams, which had previously been described [32, 33]. Increased CORT serum levels in the dams not only impact their maternal care but it can also be transferred to their pups via milk during lactation [34, 35], and the chronicity of the exposure to corticosterone through milk could also influence CORT serum levels in pups after weaning. Interestingly, we observed that only MS offspring showed high CORT concentration compared to controls: a fact associated to the longer period that MS dams were stressed when compared to the LNM group. High CORT content in mother’s milk may program the development of phenotypes involving impaired emotional processing, such as depression-like behavior and greater infant weight gain across time [36, 37].
The hyperphagia and higher body weight of MS and LNM adults are outcomes already associated with a variety of adverse early-life conditions in humans [38] that, even if they differ in intensity, chronicity, or co-occurrence, consistently alter the development of neuroendocrine systems, and impact their lipid accumulation and appetite. Our results agree with previous studies showing that only animals with MS along with social isolation exhibit higher food intake and body weight [22]; however, regarding LNM animals we were not able to distinguish the effect of each stressor in this outcome.
Our results corroborated that the HPA axis was modified in early life-stressed adult rats, although that of MS changed more drastically than that of LNM. Both stressed groups showed increased PVN Crh expression, higher in the MS than in the LNM group, which had a congruent impact on CORT serum levels that only increased in MS rats. Furthermore, only adult LNM rats subjected to the FST (LNM-FST) showed GR downregulated, which is the expected result due to the high CORT levels also observed in this group. This change contrasted with the blunted levels of CORT in MS-FST, supporting a hypersensitive or desensitized HPA axis in LNM and MS animals, respectively, most likely due to ligand-induced GR sensitivity alterations. This probably avoided a greater increase in Crh mRNA levels and higher HPA axis activity of LNM than in MS.
However, HPA axis was activated in both early-life stressed adults, favoring their greater appetite and food intake most likely due to increases in neuropeptide Y (NPY) and agouti related protein (AgRP) protein expression in the arcuate nucleus due to the high CORT levels [39, 40]. Also, since CORT participates in lipid accumulation [41], it might facilitate the elevated body weight of adults of the two postnatal stress models. The association between an impaired development of neuroendocrine axis and obesity has also been observed in humans [38, 42, 43, 44, 45, 46, 47], and explains the 17% prevalence of obesity among adults experiencing early-life stress. Our results are helpful to understand that a specific outcome, such as obesity and hyperphagia, may result from various postnatal adversities that differ in type or intensity of the inflicted stress during early periods of life.
Besides hyperphagia, MS and LNM adults also displayed a high-immobility behavior when performing the FST, which represents an impaired coping strategy to stressful situations. This high immobility was observed even when their dams’ care, HPA axis activity, and stress duration was different between the two models, which agrees with the 50% incidence of depression among humans that experienced a variety of ELS [38]. In contrast to hyperphagia and higher body weight that are induced by the combination of ELS and isolation, high immobility behavior in FST may be observed in animals subjected to MS or to isolation; interestingly, the combined stressors do not induce an additive immobility score [22, 48]. In this study, we are observing that the behavioral outcome in the FST resulted from both stressors, but we were not able to discriminate their individual effect in the experimental groups.
In MS animals, the observed depression-like behavior was associated to their unresponsive HPA axis, since neither their Crh expression nor CORT serum levels further arose in rats performing the FST. This result supported the inability of these animals to face a new energy-demanding challenge, due to impaired CORT-induced glucose availability. In contrast, LNM rats did respond to the new stressor (FST) with increased CORT serum levels even when their Crh mRNA levels remained elevated as compared to animals not subjected to the test. This result suggested a greater sensitivity of GR to CORT-induced regulation than in MS rats, and supports the assumption that ELS induces epigenetic alterations in adults’ GR gene that could alter the receptor responsiveness and HPA regulation [49].
Another explanation for the differential responsiveness of the HPA axis between MS and LNM adults to FST might be that only the amygdalar CRH pathway changed in the LNM group. LNM rats showed low Crh expression in that brain area, which could maintain CORT serum levels under basal values until adulthood, thus avoiding an exacerbated activity of the HPA axis during their development and allowing it to respond to the FST. This could be due to the amygdalar efferent CRH connections to the PVN that stimulate the HPA axis, given that the overexpression of CRH in the central nucleus of Amy is associated with impaired CORT-induced negative feedback of the HPA axis, thus inducing its hyperactivity, as well as different behavioral alterations (Gillespie et al., 2009) [50]. Since specific changes in maternal care affect the interactions between Amy and the HPA axis, those displayed by LNM dams were likely milder than those of MS. Moreover, even when LNM pups presented depression-like behavior and hyperphagia, they showed an advantage when compared to MS, which is the normal responsiveness of their HPA axis to other stressful stimuli. Also, LNM rats subjected to FST increased CORT levels that might reach the Amy and activate GR evidenced by its low expression, thus being able to downregulate the CRH pathway and its impact on HPA axis. Amygdalar Crhr1 expression also changed differentially between groups, increasing in MS and decreasing in LNM adult rats, which seemed to correlate with their HPA axis desensitization or hypersensitivity, respectively. Crhr1 upregulation in MS rats could result as a compensating change to the blocked negative feedback of the axis that maintains elevated CRH and CORT levels, which may impact the amygdalar CRH stress-responsive system. This interpretation is plausible mainly because the LNM group displayed a hypersensitive HPA axis and presented an inverse change in Crhr1 in Amy.
Crhr1 mRNA downregulation is also observed in other ELS-exposed adults, i.e. among restrain-stressed rats during early postnatal days [15, 51, 52], but not among those stressed during adulthood [51]. This supports that the time-limited CORT increase in LNM offspring, as well as the intensity and type of stress, might induce changes in Crhr1 expression, which may be representative of the brain-adaptive alterations to different types of ELS and might be associated to behavioral changes in adulthood.
In contrast to Amy, the hippocampal CRHergic system seemed deregulated only in the MS group, since its Crh expression increased when subjected to FST, avoiding a further activation of their HPA axis to the new stress. This is supported by the resulting decreased CORT serum levels after hippocampus electrical stimulation, as well as its increase by hippocampectomy or hippocampal lesions [53, 54, 55, 56, 57]. Present data align with previous studies showing that MS animals are unresponsive to acute stress such as the FST [58]. An elevated expression of GR was also specific for the MS group, which might favor their higher body weight and depression-like behavior, since other chronic stressful paradigms also developed similar symptoms in ELS adults [59]. Upregulated GR expression is interpreted as resistance mainly induced by the high CORT levels these animals had since early-life periods [59]. Furthermore, the sudden decrease in GR expression among MS-FST rats supported a deregulation of this hippocampal pathway by CORT that might upregulate Crh mRNA levels, and a specific MS-induced change in Hipp that favored the lack of response of their HPA axis to the FST, as it is thoroughly described [52, 56]. The repercussion of this hippocampal deregulated system only in MS animals resulted evident on the HPA axis by its unchanged hypothalamic PVN GR expression after exposure to FST, supporting MS rats’ inability to respond to the new challenge, reinforcing the hippocampal regulation of HPA axis sensitivity to corticosteroid feedback [56].
Hippocampal Crhr1 expression also resulted differentially altered between groups, decreasing in MS and increasing in LNM in basal conditions. This change in the MS group, associated with helplessness behavior of stressed rats [60] and a low 5-HT system functioning, is supported by the reversion induced by a fluoxetine treatment of the low Crhr1 expression and with reduced depression-like behavior and hyperphagia [61]. The decreased hippocampal 5-HT system activity in MS animals was inferred by their higher intracellular content (accumulation), along with a low metabolism of the neurotransmitter (decreased 5-HIAA/5-HT ratio) observed in the non-FST group, which were similar to the changes observed by C-FST. In contrast, the LNM group showed higher Crhr1 expression already associated to cognitive alterations [62] that are avoided with CRHR1 antagonists injections during ELS [63].
By analyzing the brain 5-HT system activity, we found that the sole FST performance was enough to induce 5-HT accumulation in Hipp and decrease serotonin pathway activity in C-FST, which resulted from 5-HT release induced by the FST in midbrain-projecting sites, such as Amy, Hipp, and PVN [64]. This could activate pre-synaptic 5-HT1A receptors in those regions favoring 5-HT accumulation. Hippocampus of the MS group also had this accumulation in rats either performing or not the FST. Decelerating 5-HT pathway in LNM rats’ Hipp was not as clear as in MS animals, but their low metabolite concentration and 5-HIAA/5-HT ratio in limbic regions supported a similar change. Specific 5-HT system alterations were found in PVN and limbic regions; but, in general, pointed out to a decreased functioning, which agreed with previous studies and supported its association with hyperphagia and depression development [65]. We did not directly prove the association between low activity of the 5-HT pathway and alterations in CRH system in the PVN and limbic regions. However, substantial evidence for this fact has been previously described [64].
We concluded that blood corticosterone elevation of stressed dams from the two paradigms differentially disturbed the HPA axis of their offspring, these results being more intense in MS than in LNM groups and seemed to be also associated to the duration of the increase. As a consequence, the HPA axis of MS adults resulted desensitized, whereas that of LNM was hypersensitized to the negative feedback effects of corticosterone. Specific differential changes in the CRH system in amygdala and hippocampus between MS and LNM adult animals might explain the variations in responsiveness of their HPA axis.
A slow activity of serotonergic neurons in the amygdala and hippocampus might contribute to the similar behavioral maladaptive outcomes that both groups of early-life stress showed in adulthood: depression-like behavior and overweight-induced hyperphagia. Moreover, early-life stressed adults’ exposure to the FST was needed for unraveling the greater vulnerability of MS animals than that of LNM when facing a new acute stressful stimulus, as the HPA axis of MS was unable to increase corticosterone levels and, in turn, generate available energy for animals to escape or to face the threat.
The datasets used and analyzed during the current study are available from the corresponding author on reasonable request.
VAA and PdG design the research study. VAA performed the research. VAA, CGL, PSC, EEC analyzed the data. PdG interpretation of data. All authors contributed to editorial changes in the manuscript. All authors read and approved the final manuscript. All authors have participated sufficiently in the work and agreed to be accountable for all aspects of the work.
All procedures were conducted with the approval of the local Ethics Committee for Animal Experimentation of the Instituto Nacional de Psiquiatría Ramón de la Fuente Muñiz (INPRFM) (CEI/C/024/2014) following the guidelines outlined by the Mexican Official Standard NOM-0620ZOO-1999.
We thank Orlando Jaimes for his technical assistance with HPLC determinations.
This research received no external funding.
The authors declare no conflict of interest.
Publisher’s Note: IMR Press stays neutral with regard to jurisdictional claims in published maps and institutional affiliations.