- Academic Editor
This is an open access article under the CC BY 4.0 license.
Background: Mitochondrial dysfunction is considered an important mechanism in the pathogenesis of various diseases. Therefore, mitochondria are currently being considered as subjects for targeted therapies, particularly, phototherapy using 5-aminolevulinic acid. This study aimed to investigate the activity of mitochondria in cells with different mutation loads. Materials and Methods: The study was conducted using 11 cybrid lines obtained from the THP-1 cell line (a human monocytic leukemia cell line) and platelets of patients with different mitochondrial mutations. Results: Our results illustrate that 5-aminolevulinic acid was metabolized equally in all cell lines, however, there was a significant decrease in mitochondrial potential, which differed among lines. Conclusions: The results of this study can be used to develop a personalized therapeutic approach based on different mitochondrial activities.
Mitochondria are double-membrane organelles that regulate many key cellular processes, including energy production, maintenance of cellular activity through cell signaling, and different metabolic pathways, which ultimately mediate cellular function [1]. In addition, mitochondria play a central role in regulating signaling activity, which determines the fate and functionality of the cell. Thus, exposure to various stress factors can result in mitochondrial damage and dysfunction, which, accordingly, leads to cell death [2]. It has been shown that mutations in the mitochondrial genome can promote mitochondrial dysfunction [3], which plays a significant role in the pathogenesis of various diseases and pathological conditions, including metabolic, cardiovascular, and oncological, among others [4]. Mitochondria-targeted phototherapy of oncological diseases, which is carried out by delivering photosensitizers specifically to the mitochondria, is a recognized strategy for increasing the effectiveness of exposure [5, 6, 7]. In mitochondria, 5-aminolevulinic acid acts as a precursor to the photosensitizer protoporphyrin IX in the heme synthesis cascade [8, 9]. Thus, in normal physiological conditions, intracellular protoporphyrin IX content is regulated by heme negative feedback. However, when 5-aminolevulinic acid is supplied from outside the cell, excess production of protoporphyrin IX may occur, which cannot be quickly utilized by the enzyme ferrochelatase into the final product, meaning it accumulates in cells at increased concentrations [10]. Thus, the accumulation of protoporphyrin IX in cells depends on the metabolic activity of mitochondria, which selectively determines its accumulation within the cell population with different dysfunction severities. Recent studies have demonstrated that 5-aminolevulinic acid may possess beneficial effects on mitochondrial metabolism and inflammatory parameters in cellular and animal models [11, 12]. In this regard, 5-aminolevulinic acid-based preparations are considered a promising therapeutic strategy in diseases associated with mitochondrial dysfunction and chronic inflammation. Currently, 5-aminolevulinic acid-based preparations are widely used in clinical practice for photodynamic therapy in theranostics as a diagnostic and therapeutic agent [13]. Concurrently, preparations based on 5-aminolevulinic acid are used to treat diseases of the lower genital tract in women [14], actinic keratosis [15], and can be used for root canal treatment [16], among others. However, the effect of 5-aminolevulinic acid on mitochondrial metabolic activity has not been studied enough. Therefore, further research should be conducted to explore the potential effect of 5-aminolevulinic acid on eliminating dysfunctional cells without the use of phototherapy. The present study aimed to investigate the effect of 5-aminolevulinic acid on the activity of mitochondria with different mutational loads.
The study included 12 cybrid cell lines of macrophage-like cells. These cell
lines were obtained by fusion monocytes of the THP-1 cell line (a human monocytic leukemia cell line) without
mitochondria (rho0), with platelets obtained from patients with different levels
of pro-atherogenic mutational loads in the mitochondrial genome [16]. The studied
11 cybrid cell lines (TC-HSM1, TC-520, TC-521, TC-522, TCI-521, TCN-521, TCP-521,
TC-HSMAM1, TC-HSMAM2, TC-HSMAM3, TC-LSM1) had the same nuclear genome but
different mitochondrial genomes. The THP-1 monocyte cell line was used as a
control. All cell lines were incubated in a 48-well plate with 5-aminolevulinic
acid (Sigma-Aldrich, St. Louis, MO, USA) at a concentration of 100
µg/mL for 18 hours [17, 18, 19], to induce protoporphyrin IX
accumulation in cultured cells. To study the effect of 5-aminolevulinic acid on
mitochondrial activity, MitoTracker™ green dye (Thermo Fisher
Scientific, Waltham, MS, USA) was added to all cell lines at a concentration of
100 µg/mL for 30 min before the start of the measurement following
the change of medium. Each cell culture was seeded into three wells of a 48-well
plate at a rate of 500,000 cells and cultured in 500 µL of RPMI-1640
(Gibco™, Waltham, MS, USA) medium at 37 °C for 48 hours.
All cell lines were validated by STR profiling and tested negative for
mycoplasma. Cells were all cultured in a humidified incubator at 37 °C
and 5% CO
The following scheme was used for each cell culture:
First well—control cells (without adding substances),
Second well—cells supplemented with MitoTracker™ green dye,
Third well—cells incubated with 5-aminolevulinic acid and MitoTracker™ green dye.
Analysis of the fluorescence intensity of protoporphyrin IX accumulation induced by 5-aminolevulinic acid was performed in all cell lines using the CLARIOstar Plus microplate reader and MACSQuant analyzer 10 flow cytometer (Miltenyi Biotec, Santa Barbara, CA, USA).
The cells were gated for each experiment based on their forward and side scatter properties (Forward versus side scatter (FSC-A vs. SSC-A) and singlets (FSC-H vs. FSC-W). Further, cells were identified by gating live cells, while dead cells were identified by being positively stained by 7-Aminoactinomycin D (7-AAD) dye (Thermo Fisher Scientific) and subsequently excluded from the analysis. The main cell population was placed in the region of interest and excluded debris. Data were analyzed using FlowJo (FlowJo LLC, Ashland, Wilmington, DE, USA). When analyzing the data with the FlowJo software package, fluorescence values were obtained in the form of normalized mean (or median) fluorescence intensity (MFI) for all studied fluorochromes. In the experiments, the fluorescence intensity values of the protoporphyrin IX fluorescence induced by 5-aminolevulinic acid were obtained by subtracting the MFI of the cells without 5-aminolevulinic acid from the MFI of the cells with 5-aminolevulinic acid. MitoTracker™ green-labeled mitochondria were identified in the population by their positive staining in the FITC-A channel (green fluorescence) (MitoTracker™ Green Fluorescent). Protoporphyrin IX autofluorescence was assessed by recording the fluorescence on the PerCP-A channel. The obtained mean (or median) fluorescence intensity values were further subjected to statistical processing.
The data were pre-normalized using the Min–Max scaling formula. The difference in normalized fluorescence intensity before and after the addition of 5-aminolevulinic acid to the cell lines was calculated as the relative change compared to the original control MitoTracker™ green fluorescence intensity value. For non-normally distributed data, statistical analysis of the multiple groups was performed using the Kruskal–Wallis test and the Conover test for multiple comparisons. The two-sample Kolmogorov–Smirnov test was used to compare two independent samples within each cell line separately (fluorescence intensity before and after the addition of 5-aminolevulinic acid).
Analysis of the protoporphyrin IX fluorescence intensity in cybrid cell lines
after an 18-hour incubation with 5-aminolevulinic acid showed no statistically
significant differences compared to the THP-1 cell line. This result indicates
that the accumulation of protoporphyrin IX, which was used as a marker of
mitochondrial activity, did not differ significantly from the control cells for
all the analyzed cybrid lines. However, significant differences in fluorescence
intensity were detected between cell lines. In particular, a significant decrease
in the level of fluorescence intensity was revealed in the cell lines TC-520 and
TC-522 compared to TC-521, p
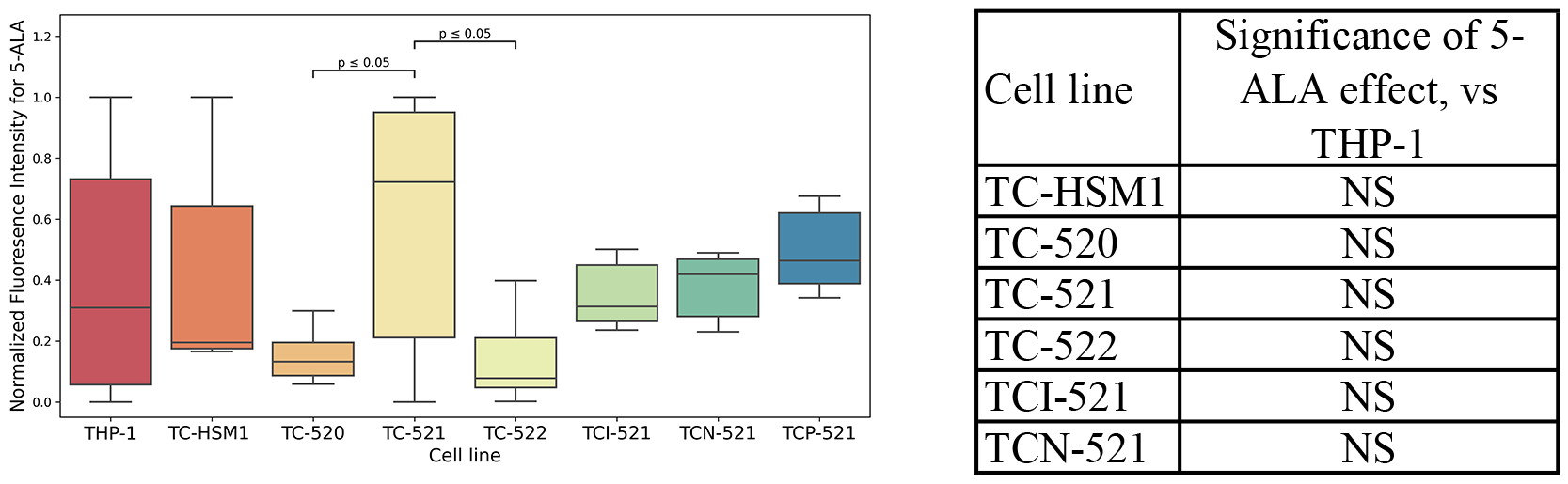
Accumulation of protoporphyrin IX in cybrid lines. NS, not significant.
MitoTracker™ green fluorescence intensity analysis was analyzed following cell incubation with 5-aminolevulinic acid using CLARIOstar Plus and showed different changes in mitochondrial potential in all cybrid lines compared to the THP-1 cell line. A comparison of the mitochondrial potential was performed between the cell lines exposed to 5-aminolevulinic acid and those that were not. A significant decrease was demonstrated in the 5-aminolevulinic acid on MitoTracker™ green fluorescence intensity in all studied cell lines. Fig. 2 demonstrates the normalized MitoTracker™ fluorescence intensity values in each cell line after incubation with and without 5-aminolevulinic acid. The effect of 5-aminolevulinic acid on the MitoTracker™ green fluorescence intensity was significantly higher in cybrid lines TC-HSM1, TC-520, TC-521, TCI-521, TCN-521, and TCP-521 compared with the THP-1 cell line.
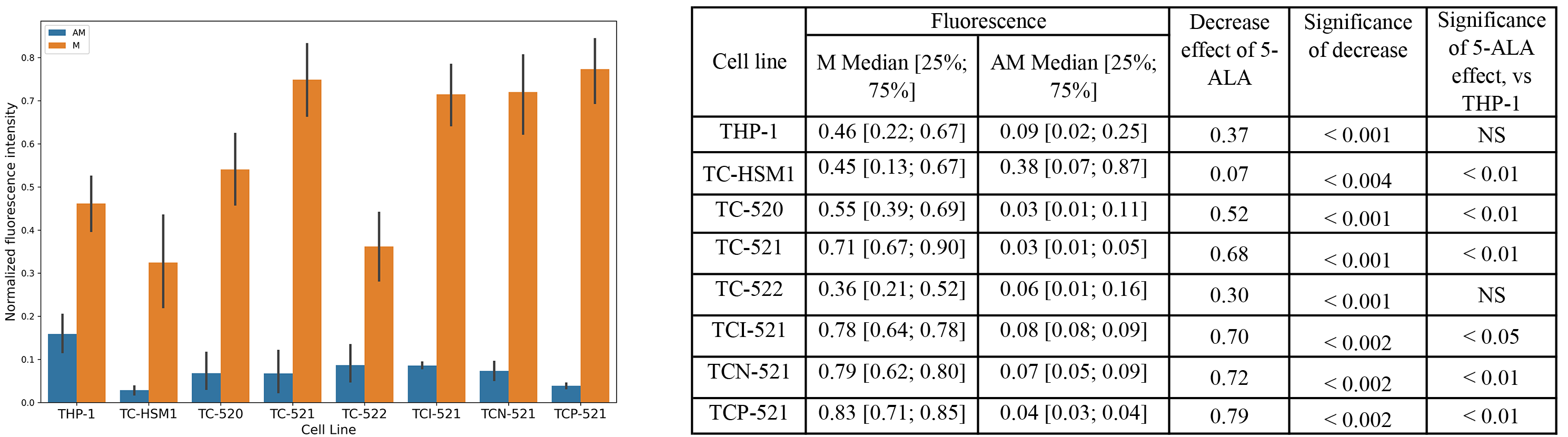
MitoTracker™ green fluorescence intensity upon incubation with 5-Aminolevulinic Acid (5-ALA) in cybrid lines compared to the THP-1 cell line, performed using CLARIOstar Plus. AM, 5-ALA and MitoTracker™ green; M, MitoTracker™ green; NS, not significant.
Analysis of the MitoTracker™ green fluorescence intensity followed 5-aminolevulinic acid incubation and was measured using the MACSQuant analyzer 10 flow cytometer. Subsequently, significant changes were observed in the mitochondrial potential for all cybrid lines compared to the THP-1 cell line. The mitochondrial potentials of cell lines exposed to 5-aminolevulinic acid and those without 5-aminolevulinic acid were compared to assess the effect of 5-aminolevulinic acid on mitochondrial activity. The effect of 5-aminolevulinic acid on MitoTracker™ green fluorescence intensity was significantly lower in all tested cybrid cell lines. Fig. 3 demonstrates the normalized MitoTracker™ fluorescence intensity values in all cybrid cell lines after incubation with and without 5-aminolevulinic acid. Notably, there was a significantly higher decrease in the effect of 5-aminolevulinic acid on MitoTracker™ green fluorescence intensity in the TC-HSMAM2, TC-HSMAM3, TCI-521, and TCN-521 cybrid lines, compared to the THP-1 cell line, which is consistent with the previous data obtained using CLARIOstar Plus.
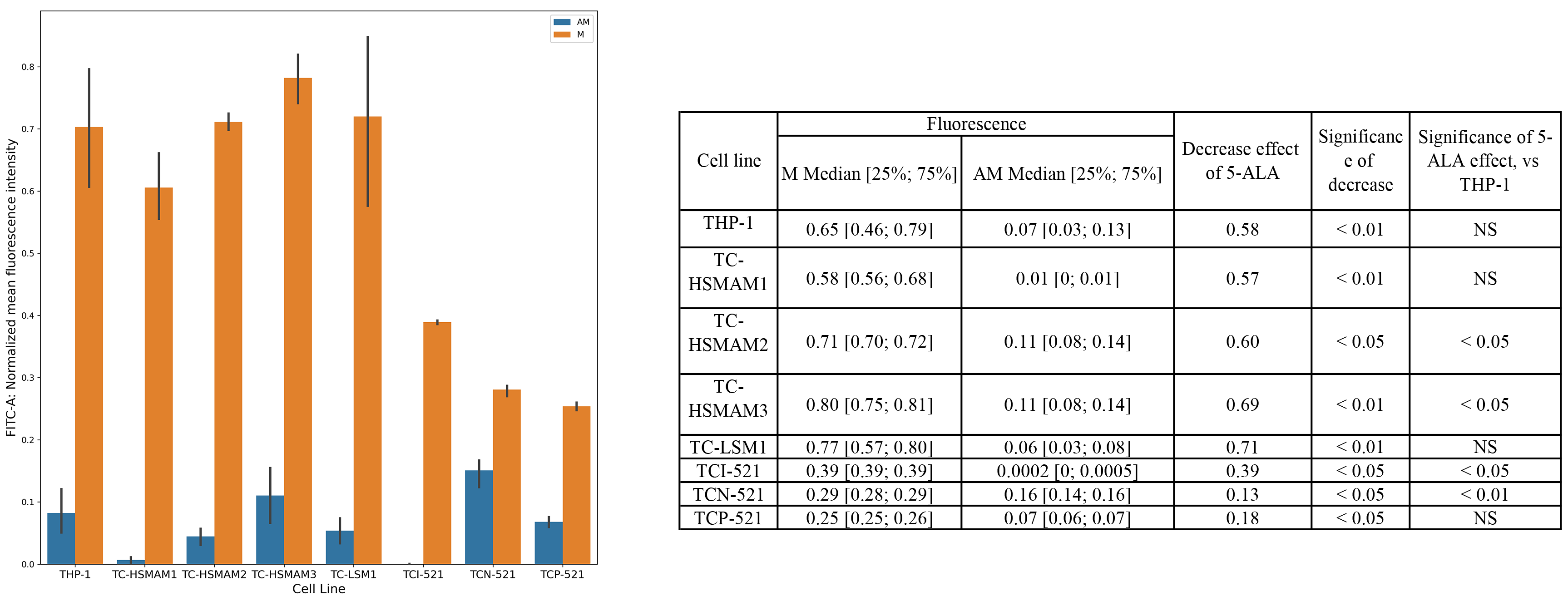
MitoTracker™ green fluorescence intensity upon incubation with 5-ALA in cybrid lines compared to the THP-1 cell line, performed using the MACSQuant analyzer 10 flow cytometer. AM, 5-ALA and MitoTracker™ green; M, MitoTracker™ green; NS, not significant.
Since the MFI values of all studied cell lines did not differ significantly compared to the THP-1 cell line, conditionally taken as the control, the results of this study indicate that most cybrid lines possess similar abilities for metabolizing 5-aminolevulinic acid and accumulating protoporphyrin IX. Moreover, the data from both the CLARIOstar Plus fluorimeter and MACSQuant 10 flow cytometer also reveal that the cybrid cell lines possess the same ability to metabolize 5-aminolevulinic acid and accumulate protoporphyrin IX. Namely, the study results demonstrated that exposure to 5-aminolevulinic acid reduced the number of mitochondria in the cybrid cell lines as well as in the THP-1 control line. This effect was reflected in a significant decrease in the MitoTracker™ green fluorescence intensity when the cells were co-incubated with 5-aminolevulinic acid. In general, these results are consistent with the results of previous studies [20]. In particular, a recent study demonstrated that 5-aminolevulinic acid therapy led to increased apoptosis of THP-1 macrophages, mediated by the production of reactive oxygen species (ROS) in mitochondria, which was also accompanied by a decrease in the mitochondrial membrane potential [21]. Another study on a model melanoma cell line showed that 5-aminolevulinic acid treatment led to the selective apoptosis and necrosis of cancer cells [22]. It should be noted that in most studies these effects were noted for photodynamic therapy, although the current study demonstrated that the effect of removing mitochondria exists without treatment by coherent light sources. Previous studies investigated the mechanism of the 5-ALA impact on the mitochondrial potential [23]. While entering the cell, 5-ALA is converted into Protoporphyrin IX (PpIX), causing intracellular PpIX accumulation that induces an increase in intracellular ROS and leads to the destruction of the mitochondrial transmembrane potential, thereby further initiating a decrease in proliferative activity and apoptosis [24]. Studies on fibroblast cell culture revealed that the toxicity of the heme synthesis intermediate PpIX can also be explained by ferroptosis; thus, targeting PpIX elevation is considered a potential strategy for the treatment of certain forms of leukemia [25]. Along with 5-ALA preparations, other mitochondria-targeting metal-based chemical agents, in particular, ruthenium complexes, can be used for photodiagnosis and therapy of various disorders, including cancer [26, 27].
This study aimed to investigate the effects of the 5-aminolevulinic acid on mitochondrial function. Currently, 5-aminolevulinic acid preparations are used in various clinical areas, including imaging tumor tissues and intraoperative monitoring during surgical interventions. Additionally, 5-aminolevulinic acid is most widely used as a diagnostic and active agent for photodynamic therapy, which is directly used to destroy superficial or cavitary tumors [28]. In addition, sonodynamic therapies, including a combination of low-intensity therapeutic ultrasound and sonosensitizing drugs, in particular 5-ALA, are being studied in different experimental models and are considered potential methods for the treatment of tumors, as well as atherosclerosis. These positive effects are achieved by inducing apoptotic effects on macrophages, as well as autophagy of tumor cells [29, 30, 31]. However, the use of 5-aminolevulinic acid as a therapeutic drug and dietary supplement for the treatment of various diseases remains controversial. Therefore, it is necessary to consider the various effects that 5-aminolevulinic acid has primarily on mitochondrial function. Since 5-aminolevulinic acid is involved in the biosynthesis of heme - the most important component of hemoglobin, increases the number and size of mitochondria in cells through activation of a transcription factor NRF-1, which in turn regulates the expression of genes involved in mitochondrial biogenesis and also increases oxygen consumption and adenosine triphosphate production in mitochondria [32, 33]. At the same time, conflicting data currently exists on the role of 5-aminolevulinic acid in protecting mitochondria from oxidative stress [34, 35]. There is evidence showing the protective properties of 5-aminolevulinic acid regarding mitochondria, which consist of the removal of ROS and increased activity of antioxidant enzymes, decreasing oxidative stress [36]. Additionally, following exposure to light, 5-aminolevulinic acid produces ROS, which ultimately leads to cell death.
Numerous studies have described the difficulty in detecting 5-aminolevulinic acid products because 5-aminolevulinic acid is a metabolic precursor of heme and does not fluoresce [37]. The heme biosynthetic pathway consists of various enzymatic steps that catalyze the conversion of 5-aminolevulinic acid to fluorescent protoporphyrin IX. The enzyme coproporphyrinogen oxidase generates protoporphyrinogen IX through oxidative decarboxylation. The next step is protoporphyrinogen IX oxidation, which is mediated by protoporphyrinogen oxidase and results in the formation of fluorescent protoporphyrin IX. Subsequently, protoporphyrin IX can either be transported outside the cell by the adenosine triphosphate-binding transporter cassette subfamily G member 2 or enzymatically modified to heme by ferrochelatase [38]. Moreover, defects in ferrochelatase—for example—mutations in this gene in humans cause protoporphyrin to accumulate in the skin, red blood cells, and liver, which in turn leads to the development of photosensitivity. Furthermore, this promotes the development of light-dependent hemolysis, strong autofluorescence of red blood cells, and subsequent toxic effects in the liver due to the accumulation of protoporphyrin IX [39]. The general metabolic pathway for heme biosynthesis, which catalyzes the conversion of 5-aminolevulinic acid to fluorescent protoporphyrin IX, has been well described. However, the exact mechanisms that lead to visible fluorescence following 5-aminolevulinic acid exposure are not fully known [40].
In the present study, two methods were used to assess the effect of 5-aminolevulinic acid on mitochondrial membrane potential, both of which obtained similar results. The macrophage-like cybrid lines were obtained based on the THP-1 cell line, which is devoid of mitochondria in monocytes and, accordingly, possesses the same nuclear genome were used as a test model in this study, in addition to platelets from patients carrying a different set of single-nucleotide variations in their mitochondrial genome. The observed results were associated with differences in the mitochondrial genome. The mitochondrial potential indicator calculated through the fluorescence intensity of the mitochondrial dye MitoTracker green (normalized mean (or median) fluorescence intensity) was used to estimate the number of mitochondria. Additionally, the metabolic ability of the cells to process 5-aminolevulinic acid and its accumulation in the form of protoporphyrin IX was the parameter used to indicate the normalized mean (or median) fluorescence intensity owing to its ability to reflect the actual autofluorescence of protoporphyrin IX.
The results of the current study are consistent with previous studies and suggest that 5-aminolevulinic acid has the potential to improve both mitochondrial metabolism and its functions. The biological effects of 5-aminolevulinic acid have been widely investigated using various cellular models. Indeed, it was demonstrated in a murine proximal tubular epithelial cell model that treatment with 5-aminolevulinic acid ameliorated the severity of oxidative stress and apoptosis [41]. Anti-oxidant properties of 5-aminolevulinic acid were also confirmed in a cardiomyocyte hypertrophy cell model [42]. Previous experiments using a RAW 264.7 macrophage model also determined the inhibitory effects of 5-aminolevulinic acid on lipopolysaccharide-induced inflammatory cytokine secretion and ROS production [43]. In addition, 5-aminolevulinic acid exhibited an anti-inflammatory effect in a transgenic rat animal model by reducing macrophage activation and the expression of inflammatory mediators [44]. Moreover, it was shown that 5- 5-aminolevulinic acid prevented a decrease in mitochondrial DNA copy number and abnormalities in mitochondrial morphology commonly associated with experimental stress factors, increased antioxidant transcription activity of mitochondria, while also suppressing the expression of inflammatory genes and promoted anti-inflammatory polarization of macrophages [11, 12]. A recent transcriptome study also revealed differentially expressed genes associated with DNA damage, oxidative stress, and mitochondrial dysfunction in HepG2 cells after exposure to 5-aminolevulinic acid, thereby demonstrating the possible toxicity of 5-aminolevulinic acid treatment [45]. Therefore, given the promising results of both the previous and current studies as well as the conflicting results of recent research, the use of 5-aminolevulinic acid-based therapies can be considered a potential treatment. The obtained data on the presence of mitochondria-associated effects by 5-ALA on the studied cell lines can be used to further study the development of therapeutic strategies without requiring the use of photodynamic therapy. However, future studies are needed to evaluate the effectiveness and safety of 5-ALA therapy.
The results of the current study showed that exposure to 5-ALA caused a decrease in the fluorescence intensity of MitoTracker™ green in cybrid cell lines with different levels of the mitochondrial genome, compared to the THP-1 cell line. Given the currently available conflicting results on the effects of 5-ALA, further research is necessary to elucidate the mechanisms of its action. However, the obtained data can be used to develop new therapeutic strategies for treating diseases associated with mitochondrial dysfunction.
Data are available from the corresponding author upon request.
AMM and ANO designed the research study. YVM, TVT and VRC performed the research. AMM, TVK and DGK analyzed the data. YVM and TVK wrote the manuscript. All authors contributed to editorial changes in the manuscript. All authors read and approved the final manuscript. All authors have participated sufficiently in the work and agreed to be accountable for all aspects of the work.
This work was performed in accordance with the Declaration of Helsinki of 1975 and its revised version of 2013. The study protocol # 5 was approved by the Local Ethics Committee of the Petrovsky National Research Centre of Surgery at May, 05, 2022.
Not applicable.
This work was funded by the Russian Science Foundation, Grant # 22-25-00190.
The authors declare no conflict of interest.
Publisher’s Note: IMR Press stays neutral with regard to jurisdictional claims in published maps and institutional affiliations.