Academic Editor: Alessandro Poggi
Obesity and osteoporosis are global health problems characterized by high rates of prevalence and mortality due to complications. As people with visceral obesity age, the adipogenic differentiation of bone marrow mesenchymal stem cells (BMSCs) increases, and adipocytes become the predominant stromal cells in the bone marrow microenvironment, which hinders the physiological regeneration and mineralization of bone tissue. Primary and secondary osteoporosis remain severe progressive diseases. Both osteoporosis and obesity are associated with microRNAs (miRNAs) that induce adipogenesis and osteoresorption. This review presents analyses of the roles and clinical potential of miRNAs in the epigenetic control of BMSC differentiation and the formation and function of osteoclasts in osteoporosis with and without obesity. Understanding the fine-tuned regulation of the expression of genes critical for the balance of osteogenesis/osteolysis processes may provide hope for the development of effective and safe osteoporosis therapies in the future.
Obesity and osteoporosis are characterized by high prevalence and mortality rates due to associated complications; therefore, they represent global health problems. Osteoporosis is a systemic, multifactorial disease characterized by the loss of bone mass, impaired metabolism, and disrupted bone tissue microarchitecture, which are accompanied by an increase in skeletal fragility and fracture injuries caused by minimal trauma [1].
The effects of obesity on bone health are very controversial. Increased body
weight due to obesity increases the mechanical load on the bones, stimulating
bone growth. In subcutaneous and visceral adipose tissue, aromatase synthesizes
estrogen, which inhibits osteoclast activity and increases bone mass [2].
Therefore, an increased body mass index (BMI) value in postmenopausal women is
associated with estrogen production and increased osteoblast activity, which
generally reduces the risk of fractures. However, a critical increase in adipose
tissue has been shown to exert a negative effect on bone mass [3]. Thus,
osteoporosis is exacerbated by proinflammatory cytokines with proresorptive
properties (tumor necrosis factor (TNF)-
With increasing age, bone marrow mesenchymal stem cells (BMSCs) exhibit a decreased capacity for differentiation into osteoblasts, and adipogenic differentiation increases with an increase in the volume of yellow bone marrow. Furthermore, under visceral obesity conditions, BMSC differentiation into adipocytes is promoted, which has a negative effect on bone strength [5, 6, 7, 8]. Thus, with increasing age against a background of morbid obesity, adipocytes become the predominant stromal cells in bone marrow [9, 10].
The modern view of bone formation and resorption is based on epigenetic regulation mechanisms [11]. The involvement of microRNAs is considered key to the control of BMSC gene expression [12, 13].
MicroRNAs are small noncoding RNAs with lengths of 19–22 nucleotides, and they control biological and pathological processes, including organogenesis, apoptosis, cell proliferation, and differentiation [14]. MicroRNAs bind to specific sequences in the 3’ and 5’ untranslated regions (UTRs) of their target mRNAs to induce translational repression and mRNA deadenylation and decapping [11, 14]. Studies have shown that microRNAs are stable outside the cell and do not degrade when held at room temperature for up to 24 hours or at –80 °C for up to 9 months or after thawing in plasma/serum eight times [15]. Over the past 20 years, the number of publications in the PubMed database (https://pubmed.ncbi.nlm.nih.gov) retrieved with the search keyword “microRNA and cell differentiation” has increased by 780 fold (reaching 1557 articles in 2021). However, the roles of microRNAs in the autocrine and paracrine regulation of BMSC differentiation, including the development of osteoporosis in obesity, have not been adequately studied.
This review is dedicated to analyzing the roles and clinical potential of microRNAs in the epigenetic control of BMSCs and osteoclast differentiation in osteoporosis with obesity.
In the differentiation of mesenchymal stem cells, a cascade of events causes phenotypic and metabolic changes. Decreased expression of stem cell genes and the activation of genes associated with mature phenotypic function are the first steps in the cascade, and they lead to cellular morphological changes. Several studies have shown that factors and signaling pathways that stimulate adipogenesis simultaneously inhibit osteogenesis [16]. The reciprocal balance of the transcriptome between adipogenesis, osteogenesis, and chondrogenesis is essential for skeletal tissue development and the maintenance of homeostasis in a mature organism [17].
In healthy bones, the continuous resorption of old bone mainly by osteoclasts and the formation of new tissue by osteoblasts occur. Osteoclasts originate from the hematopoietic lineage [18] and monocyte/macrophage-derived osteoclast precursors [19]. Osteoblasts originate from BMSCs and can differentiate into adipogenic clones [20]. The microenvironment strictly controls BMSC differentiation, and imbalanced differentiation leads to a higher rate of adipogenic MSC differentiation, which is detrimental to osteogenesis [17, 20, 21].
An increase in adipose tissue mass leads to serious effects on the bone marrow
microenvironment due to secreted factors. That is, the secretion of
proinflammatory cytokines, adipokines, free fatty acids, exosomes, and microRNAs
by adipocytes stimulates osteoclasts and promotes increased bone resorption [22].
In addition, obesity contributes to a disturbance/alteration in adipokine
secretion; therefore, the secretion of adiponectin decreases, and the production
of leptin and proinflammatory factors increases. Studies of transgenic mice
harboring the human adiponectin gene demonstrated the stimulatory effect of
adiponectin on bone formation through the activation of the Wingless-type MMTV
integration site (WNT)/
Increased TNF-
Simultaneously, TNF-
Obesity is associated with increased osteoblast production of RANKL, which
regulates osteoclast differentiation, proliferation, survival, and apoptosis.
Thus, adipose tissue secretes M-CSF, which induces RANKL-mediated bone resorption
by triggering the PI3K/AKT/NFkB signaling cascade, activating NFATC1, or
upregulating the expression of the transcription factors HIF-1
Osteoprotegerin (OPG), which is a member of the TNF receptor superfamily (TNFRSF11B), is secreted by osteoblasts. It is a RANKL decoy receptor that blocks the RANKL/RANK interaction [31, 32]. An increased OPG level with age is considered a homeostatic response to limit bone resorption [33]. It should be considered that high insulin levels in obesity interact with insulin receptors on osteoblasts, blocking the production of OPG and increasing the secretion of osteocalcin [34]. The presence of obesity and type 2 diabetes is associated with the active production of oxidized lipids and carbohydrates in addition to damaged proteins, leading to the formation of enhanced glycation end products (AGEs). AGEs interact with the AGE receptor (RAGE) and trigger a cascade of intracellular responses (NFkB, AP-1, CREB, STAT3, and NFAT), leading to the development of inflammatory responses [35]. The accumulation of AGEs in bone tissue leads to the development of pathological conditions associated with low bone density and osteoporosis [36]. Considering these finding, the triggering of inflammatory responses is a link between metabolic disorders, osteogenesis and bone resorption.
The intracellular mechanisms that determine MSC fate in the context of adipogenic and osteogenic differentiation are under active investigation [17]. The processes of MSC differentiation into adipocytes and osteocytes in vitro can be conditionally classified into two stages: (1) clone predetermination (the commitment stage) from an MSC to a committed progenitor and (2) maturation (the terminal differentiation stage) from the progenitor to a cell with a mature phenotype [16, 21].
Numerous studies have shown that the major signaling pathways involved in
regulating MSC lineage commitment are the transforming growth factor-beta
(TGF
Adipogenic differentiation is associated with increased production of cyclic
AMP, leading to the phosphorylation of the transcription factor
CCAAT/enhancer-binding protein (C/EBP). Upon activation, C/EBPB binds to
regulatory elements and stimulates the transcription of C/EBPA and peroxisome
proliferator activated receptor gamma (PPAR
Thus, adipogenesis and osteogenesis are regulated by complex factor cascades, and the role of RNA-dependent epigenetic regulation is extremely important. MicroRNAs form interaction networks that provide additional control over biological processes. In regenerative medicine, MSCs are used in alternative therapies for the treatment of obesity [38, 39] and bone regeneration [17]. Understanding the regulatory pathways involved in cell commitment and investigating the factors that regulate the balance between osteogenic and adipogenic differentiation in MSCs are of great importance for the theoretical understanding of the pathogenesis of osteoporosis in obesity and will enable the identification of molecular targets for the treatment of osteoporosis in the future.
MicroRNAs may control the transition processes between the osteogenic and adipogenic differentiation of MSC-BMs [17]. An analysis of data reported in the scientific literature showed increases in the levels of miR-222, miR-140-5p and miR-142-5p, miR-142-3p [40], miR-143-3p [41], miR-217 [42], and miR-16 [43] in serum/plasma and an increase in miR-221/222 only in women [44].
In an animal model of obesity, the levels of miR-211, miR-130b, miR-188, and miR-128 were increased [45, 46, 47, 48] (Table 1, Ref. [49, 50, 51, 52, 53, 54, 55, 56, 57, 58, 59, 60, 61, 62, 63]). Several microRNAs are known to induce BMSC adipogenesis by inhibiting the activity of the transcription factor RUNX2, which is involved in osteoblastogenesis. These microRNAs include miR-204, miR-211, miR-217, miR-221, and miR-222.
№ | miRNAs and target cells | Target gene | Signaling pathway | Cell effect | miRNA levels in bone tissue | References |
1 | RUNX2 | activation of PPAR pathway | enhancement of adipogenic | - | [49] | |
miR-211 | (gene PPAR |
differentiation | ||||
rat BMSCs, ST2 cells | ||||||
2 | RUNX2 | downregulation of MAPK/ERK pathway | suppression of osteogenic differentiation | - | [50] | |
rat BMSCs | ||||||
3 | RUNX2 | activation IGF-1/ERK pathway | suppression of osteogenic differentiation | [51] | ||
miR-222-5p | ||||||
rat BMSCs with high glucose | ||||||
4 | activation of PPAR |
enhancement of adipogenic | [52] | |||
mouse and human BMSCs | differentiation | |||||
5 | SIRT6 | - | enhancement of adipogenic differentiation and suppression of osteogenic differentiation | [53] | ||
C2C12 cell line | ||||||
6 | VEGF | downregulation of VEGF pathway | [54] | |||
human BMSCs | ||||||
7 | TGFBR1 | increased mRNA gene C/EBPs | enhancement of adipogenic | - | [55] | |
mouse BMSCs in adipogenic medium | differentiation | |||||
in vitro | ||||||
8 | BMP2 | downregulation | inhibition of osteogenic commitment | - | [56] | |
human BMSCs | of BMP2-mediated pathway | |||||
9 | WNT5A | downregulation of WNT pathway | enhancement of adipogenic | [57] | ||
differentiation | ||||||
human BMSCs | activation of pathway | |||||
PPAR |
||||||
10 | Osx | - | suppression of osteogenic differentiation | [58, 59, 60] | ||
human BMSCs | ||||||
MC3T3-E1 cell line | ||||||
11 | HDAC9 | increased mRNA of gene PPAR |
age-related bone loss | [61] | ||
in vitro | RICTOR | enhancement of adipogenic | ||||
differentiation | ||||||
12 | ETS1 | downregulation of RUNX2 pathway | suppression of osteogenic differentiation | [62] | ||
MC3T3-E1 cell line | ||||||
13 | - | downregulation p21 pathway (gene Oxr1) | enhancement of adipogenic differentiation and suppression of osteogenic | [63] | ||
in vitro/in vivo | downregulation of FOXO pathway (gene FoxO3) | differentiation | animal model | |||
RUNX2, runt-related transcription factor 2; SIRT6, sirtuin 6; VEGF- vascular endothelial growth factor; TGFBR1- transforming growth factor beta receptor 1; BMP2, bone morphogenetic protein 2; WNT5A, Wnt family member 5A; OSX, osterix; HDAC9, histone deacetylase 9; RICTOR, RPTOR-independent companion of MTOR complex 2; ETS1, ETS proto-oncogene 1, transcription factor; FoxO3, forkhead box O; IGF-1, insulin-like growth factor 1; ERK, extracellular signal-regulated kinase. |
Notably, high miR-204 and miR-211 expression in BMSCs induces adipogenesis by
decreasing the activity of the transcription factor RUNX2 (Table 1).
RUNX2 deficiency leads to increased adipogenesis. As a result, researchers have
observed increases in the expression of PPAR
Notably, miR-130a can regulate BMSC differentiation in both the adipogenic or
osteogenic directions. Bioinformatics analysis revealed that Smurf2 and
PPAR
Zhu et al. (2017) [50] showed that miR-217 expression was negatively associated with bone formation and the osteogenic differentiation of BMSCs in rats because this miRNA inhibited RUNX2 activity. This molecular mechanism was mediated by the ERK and p38 MAPK signaling pathways (Fig. 1).
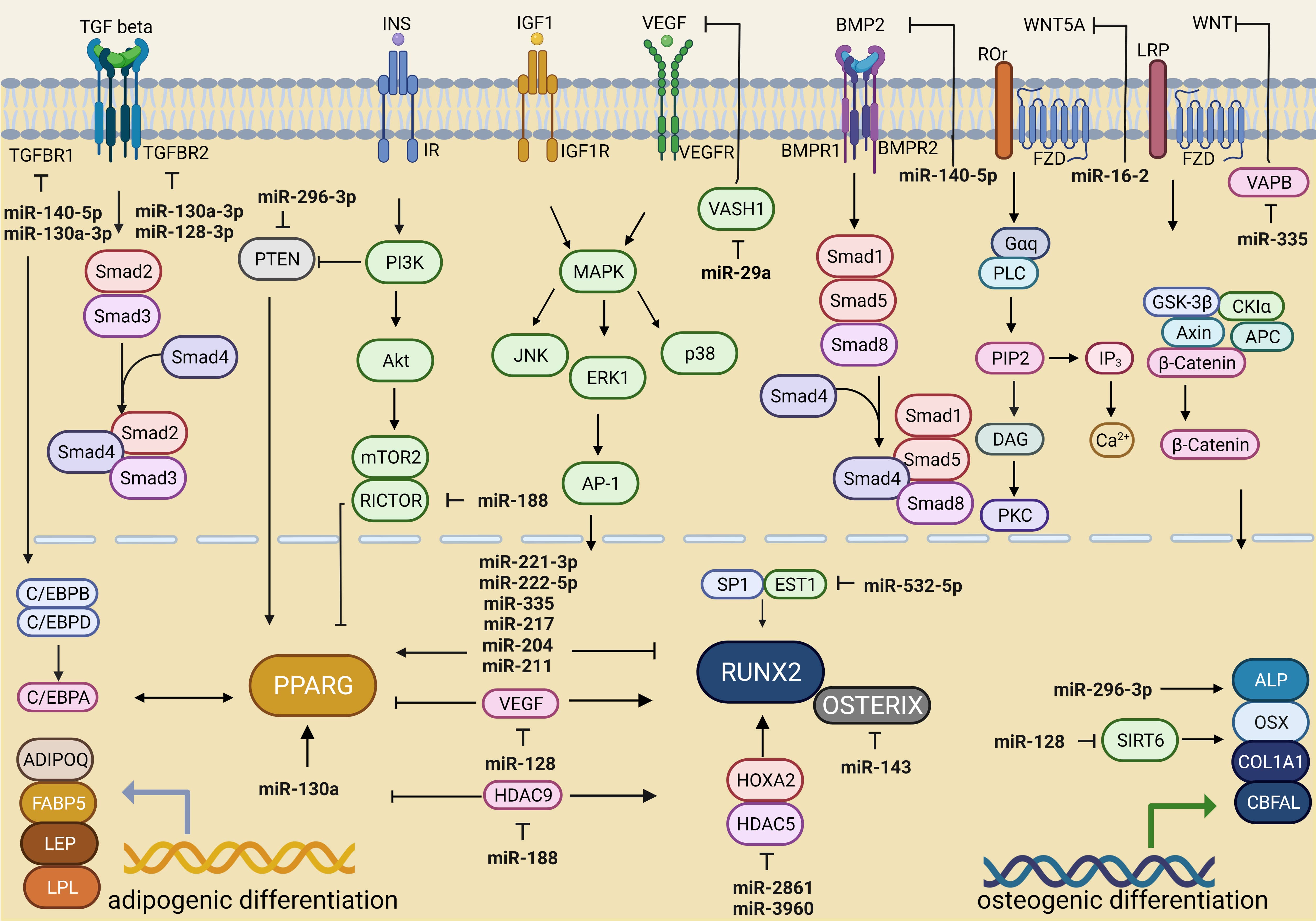
miRNA-dependent regulation of signaling pathways associated with adipogenic and osteogenic differentiation. Created with BioRender.com.
The ERK signaling pathway is involved in the insulin-like growth factor receptor (IGF)-1-mediated osteogenic differentiation of BMSCs. Therefore, increased expression levels of miR-221-3p and miR-222-5p against a high-glucose concentration background were associated with the suppression of BMSC osteogenic differentiation via the IGF-1/ERK pathway [51] (Table 1) (Fig. 1).
In patients with osteoporosis, the miR-128 level was shown to be significantly
increased compared to that in patients without osteoporosis [53] (Table 1). The
authors suggested that miR-128 inhibits osteoblast differentiation by
suppressing sirtuin (SIRT) 6 expression, thus accelerating osteoporosis
development (Fig. 1). Studies of C2C12 cells transfected with short interfering
RNA against SIRT6 (si-SIRT6) showed decreases in osteocalcin mRNA and
protein expression, alkaline phosphatase (ALP) activity, and collagen I
type-
TGF-
In another study, miR-140-5p was shown to reduce the activity of molecules involved in the BMP signaling pathway, including BMPR2, BMPR1B, and SMAD5, and prevent the osteogenic differentiation of undifferentiated hMSCs [56] (Table 1) (Fig. 1).
In patients with osteoporosis, the expression level of miR-16-2 was
significantly increased in bone tissue and was associated with bone resorption
factors and osteoblast differentiation process inhibition [57] (Table 1). The
WNT5A gene is a target of miR-16-2-3p (miR-16-2*), and this mRNA–miRNA
interaction blocks WNT signaling and leads to adipogenesis induction mediated
through PPAR
High expression of miR-143 has been found in BMSCs during osteoporosis and has been associated with adipogenesis [58, 67]. A common target between miR-143 and miR-145 is the OSX gene, which is critical for BMSC osteogenic differentiation [59, 60, 67] (Table 1).
An increase in the level of miR-188 in BMSC mediates bone loss and promotes
adipocyte accumulation during aging [61]. In vitro studies showed that
miR-188 inhibited histone deacetylase 9 (HDAC9) and
rapamycin-insensitive companion of mammalian target of rapamycin
(RICTOR) expression and upregulated PPAR
The development of obesity was associated with elevated levels of miR-143 in serum and adipose tissue [70]. Moreover, miR-204 [71], miR-296 [52], and miR-128 [72] were increased in visceral adipose tissue in the context of obesity. Specifically, the authors showed that in obesity, miRNA-21 expression was increased in visceral and subcutaneous adipose tissue but was decreased in serum [73, 74] (Table 1).
However, in women, miR-130 [52] and miR-145-5p levels were found to be decreased in subcutaneous adipose tissue [75, 76], indicating obesity (Table 1). The level of miR-145 was found to be suppressed against the background of chronic subclinical inflammation in the adipose tissue of the greater omentum in obese patients with/without type 2 diabetes [77] and in serum in the contexts of obesity [76] and osteoporosis [78] (Table 1).
In addition, low miR-532-5p expression was associated with obesity [79]; in contrast, high expression was found in individuals with low bone mineral density [62, 80]. The target of miR-532-3p is the transcription factor ETS proto-oncogene 1 (ETS1) (Fig. 1). During osteoblast differentiation, synergistic interaction between the transcription factors ETS1 and transcription factor specificity protein 1 (SP1) promotes RUNX2 expression [81] (Table 1). The overexpression of miR-532-3p in MC3T3-E1 cells indirectly reduced the expression of osteogenic markers (collagen 1, RUNX2, ALP, osteopontin, and osteocalcin) and resulted in the loss of bone mineral density [62] (Table 1). Therefore, considering these recent studies, we propose that a decrease in miR-532-5p expression against the background of obesity may contribute to the maintenance of bone integrity (Table 1).
These studies indicated that the aforementioned microRNAs (miRNA-204, miRNA-211, miRNA-217, miRNA-221 miRNA-222, miRNA-130/130a, miRNA-296, miRNA-140, miRNA-16-2/16-3p miRNA-21, miRNA-143, miRNA-188, and miRNA-128) may control osteoresorption processes by activating BMSC adipogenesis, inhibiting key gene/transcription factor expression against a background of obesity and dysregulating normal osteogenesis (Table 1).
MicroRNAs may exert direct impacts on osteoblast formation by regulating transcription factors and maintaining bone tissue homeostasis.
An increase in miR-296 expression can trigger the osteogenic differentiation of MSCs (osteoblastogenesis) and decrease the activity of the transcription factor Pten, which is critical for BMSC differentiation into adipocytes [82] (Table 2, Ref. [82, 83, 84, 85, 86, 87, 88, 89]). In a human osteoblast cell line (hFOB 1.19 cells), the overexpression of miR-296 contributed to a significant increase in matrix mineralization, ALP activity, osteocalcin expression, and increased core-binding factor al (Cbfal) expression [83] (Table 2).
№ | miRNAs and target cells | Target gene | Signaling pathway | Cell effect | miRNA levels in bone tissue | References |
1 | PTEN | negative regulation of PPAR |
enhancement of osteogenic differentiation | [82] | ||
BMSCs | ||||||
2 | - | increased mRNA for genes CBFAL | positive effect on differentiation, migration, invasion and proliferation of osteoblasts, negative effect on cell apoptosis | [83] | ||
hFOB 1.19 cell line | OSX | |||||
COL-1 | ||||||
3 | HDAC5 | activation of transcription factor RUNX2 | enhancement of osteogenic differentiation | [84, 85] | ||
miR-3960 | HOXA2 | |||||
ST2 cell line | ||||||
4 | VAPB | activation of | enhancement of osteogenic differentiation, bone fracture repair | - | [86, 87] | |
in vitro | Wnt/ | |||||
in vivo | pathway | |||||
5 | RUNX2 | - | enhancement adipogenicof osteogenic differentiation | [86, 88] | ||
human BMSCs | ||||||
6 | VASH1 | - | stimulation of angiogenesis and osteogenesis | - | [89] | |
BMSCs | ||||||
in vitro | ||||||
in vivo | ||||||
RUNX2, runt-related transcription factor 2; OSX, osterix; PTEN, phosphatase and tensin homolog deleted on chromosome 10; HDAC5, histone deacetylase 5; HOXA2, homeobox A2; VapB, vesicle-associated membrane protein-associated protein B/C; VASH1, vasohibin 1; CBFAL, core-binding factor al; Col-1, collagen type I. |
The mechanism of action of miR-2861 and miR-3960 in the same locus is related to increased RUNX2 transcription factor expression and repressed activity of its inhibitors histone deacetylase 5 (HDAC5) and homeobox A2 (HOXA2), leading to osteoblast differentiation [84, 85] (Table 2).
Low levels of miR-2861 have been found in the bone tissue of patients with osteoporosis [85] (Table 2) and in the subcutaneous adipose tissue of obese patients [90].
A recent study by Abazari et al. (2020) [91] showed that miR-2861-transduced induced pluripotent stem cells (iPSCs) exhibited high viability, mineralization levels, alkaline phosphatase activity, calcium contents, and expression of genes related to bone formation compared with untransduced iPSCs. The combined use of miR-2861-transduced iPSCs with a poly(lactic-co-glycolic acid) (PLGA) nanofiber scaffold and an artificial extracellular matrix had a positive effect on the osteogenic differentiation potential of iPSCs; therefore, this strategy can be used in bone tissue engineering.
No information was found on the dynamics of changed miR-3960 levels against the background of obesity. However, in obese patients with type 2 diabetes, the exosomal level of miR-3960 was significantly higher than those in patients with type 2 diabetes and healthy donors [92]. Previously, Ding et al. (2015) [93] demonstrated a decrease in hsa-miR-3960 in the serum of patients with type 2 diabetes.
miR-335 is the most highly expressed microRNA in BMSCs [94], but its expression
decreases during the osteogenic differentiation of MSCs [95]. Hu et al.
(2021) [86] demonstrated that the introduction of extracellular vesicles
containing miRNA-335 derived from BMSCs promoted osteoblast differentiation in
cell models and recovery after fractures in an animal model by suppressing the
expression of the vesicle-associated membrane protein-associated protein
B (VapB) gene (Table 2). The overexpression of VapB inactivates the
WNT/
Associations have been established between the loss of microRNA-29a and markers of oxidative stress (8-hydroxydeoxyguanosine (8-OHdG), DNA hypermethylation (5-methylcytosine (5mC)) and osteoblast aging in human osteoporosis because these processes are regulated by oxidation resistance protein-1 (Oxr1) and forkhead box O (FoxO3) gene products [63] (Table 1).
miR-29a in exosomes derived from MSC-BMs promoted angiogenesis and osteogenesis in vitro and in vivo. The vasohibin 1 (VASH1) gene, which is considered a negative regulator of angiogenesis, was identified as a direct target of exosomal miR-29a [89] (Table 2). Another study showed that miR-29a overexpression disrupted adipogenesis [96]. However, miR-335, miR-218, and miR-29a levels increased with obesity [97, 98, 99].
Thus, increasing the levels of miR-296-3p, miR-2861, miR-3960, miR-335-5p, and miR-29a in the MSC-BM microenvironment may normalize/activate osteogenesis processes and prevent bone loss in osteoporosis.
Osteoporosis is characterized by an increase in osteoclastogenesis associated with bone resorption. Against a background of obesity, a significant fraction of miRNA influences the rate of osteoclastogenesis, which leads to excessive osteolysis. Inflammation plays an important role in the induction of osteoclast differentiation [28].
Key microRNAs involved in osteoclast differentiation have been identified in RAW264.7 and 293T mouse monocyte/macrophage lines. The expression of miR-199b-5p, miR-10a-5p, miR-10b-5p, miR-24-1-5p, miR-223-3p, and miR-338-3p was suppressed, whereas miR-291a-5p, miR-125b-1-3p, and miR-291b-5p expression was enhanced (Table 3, Ref. [100, 101, 102, 103, 104, 105, 106, 107, 108]) (Fig. 2).
№ | miRNAs and target cells | Target gene | Signaling pathway | miRNA levels in bone tissue | References |
1 | IΚK |
activation of | [100, 101] | ||
RAW264.7, 293T cell line | NF-κB pathway | ||||
2 | SIRT1 | activation of NF-kB pathway | [102] | ||
bone marrow macrophages | osteoporosis-model mice | ||||
3 | IGF2 | activation of osteoclastogenesis | [103] | ||
CD14+РВМС cell line | |||||
4 | - | activation of osteoclastogenesis increased NFATc1, c-Fos, TRAP mRNA | - | [104] | |
RAW264.7, THP-1 cell line | |||||
5 | - | - | [105] | ||
RAW264.7, THP-1 cell line | |||||
6 | TNFRSF1B | activation of NF-kB pathway | [106, 107] | ||
RAW 264.7 cell line | activation of osteoclastogenesis | ||||
7 | Cd226 Srgap2 | activation of osteoclastogenesis | - | [108] | |
ex-miR-145 | |||||
mice BMSСs | |||||
I |
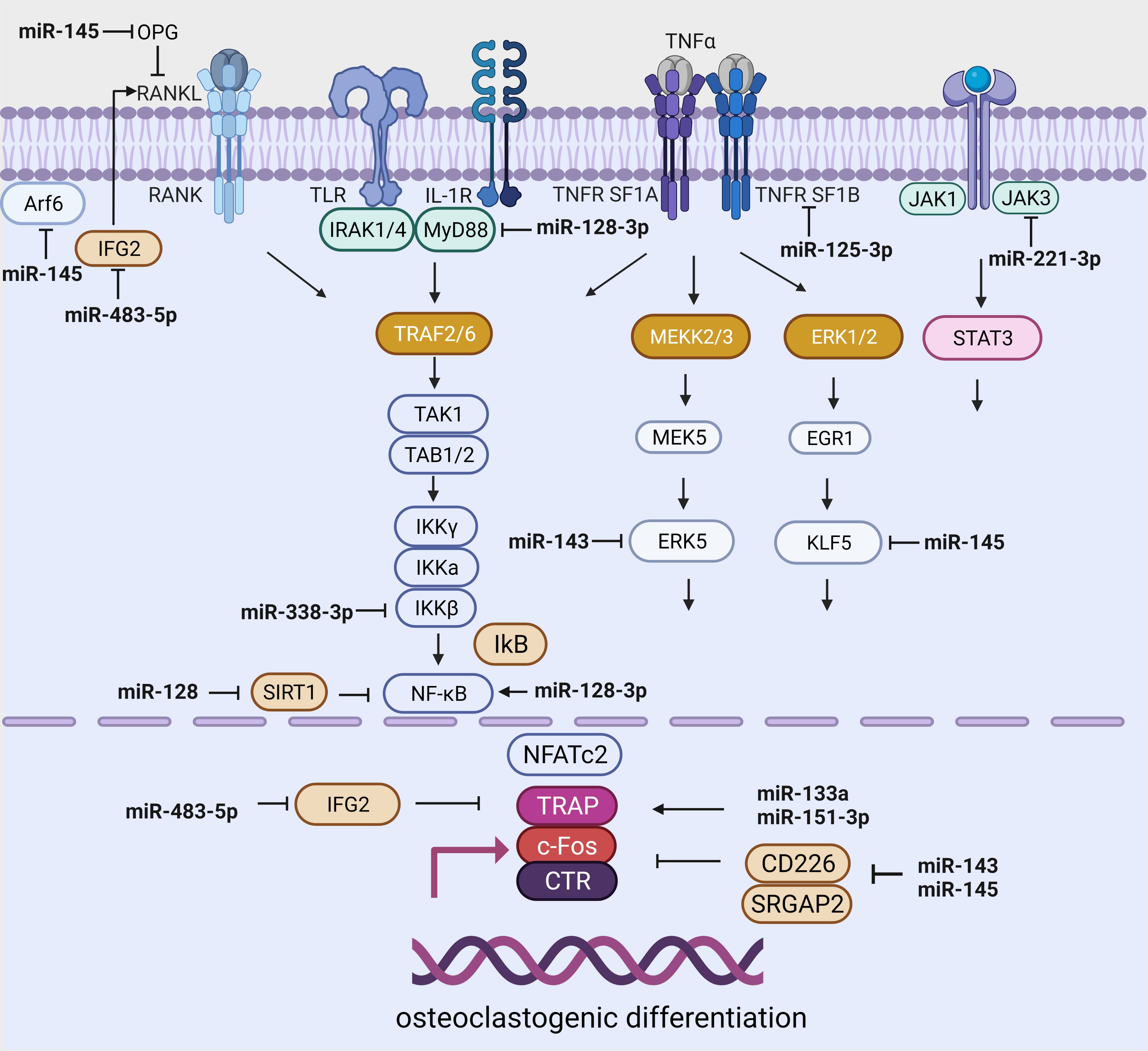
miRNA-dependent regulation of signaling pathways related to osteoclast differentiation. Created with BioRender.com.
Further studies showed that the overexpression of miR-338-3 restricted
osteoclast formation by regulating IKK
The level of miR-338-3p is increased in the serum of women with postmenopausal osteoporosis; however, it is decreased in obese patients [100, 101] (Table 3).
Numerous studies have shown that miR-128 is involved in the regulation of
signaling pathways associated with osteoclastogenesis [102, 109] and with reactive
oxygen species, inflammation, and fibrosis in many tissue types [109]. In HK2
cells, miR-128-3p was shown to inhibit apoptosis and inflammation by targeting
the TGFBR2 gene [110]. Another target of miR-128-3p is MyD88,
which activates NF-
In contrast, Shen (2020) [112] showed that the miR-128/SIRT1/NF-
High expression of miR-483-5p and low levels of IGF2 were found in blood serum and bone tissue in patients with osteoporosis. Moreover, an increase in miR-483-5p promoted the differentiation of CD14+ PBMCs into osteoclasts [103] (Table 3) (Fig. 2). In recent studies, miR-483-5p was positively correlated with the BMI value and associated with a poor prognosis and an elevated risk of developing diabetes mellitus and cardiovascular disease [113, 114].
In women with postmenopausal osteoporosis, the levels of miR-133a and miR-151a-3p were increased in blood serum and negatively correlated with bone mineral density [104, 105] (Table 3). The overexpression of miR-133a and miRNA-151a-3p activated the RANKL-induced differentiation of RAW264.7 and THP-1 cells (murine monocyte/macrophage lines) into osteoclasts (Fig. 2).
In subcutaneous adipose tissue, an increase in miR-133a levels was detected against a background of obesity [115]. However, Oliveira et al. (2020) [116] showed that miR-133a levels were reduced in subcutaneous adipose tissue. Specifically, the microRNA-151a-3p level was reduced in the subcutaneous adipose tissue of obese women [74] and in the serum of obese patients [117] (Fig. 2).
The target of miR-125a-5p is the tumor necrosis factor ligand superfamily member 1B (TNFRSF1B) gene. The overexpression of miR-125a-5p indirectly inhibited osteoclast differentiation and functional activity by decreasing the expression of TNFRSF1B [107] (Table 3). MiR-125a-5p was found in serum MSCs derived from the bone marrow of patients with osteoporosis [118, 119] and was actively increased in osteoblasts and osteoclasts [106] in subcutaneous adipose tissue in obesity and type 2 diabetes [120], and these findings were associated with bone fragility in women [121] (Fig. 2).
The expression of miR-143-145 was significantly increased in the MSCs of postmenopausal women compared with that in the MSCs of premenopausal women. The authors demonstrated that miR-143/miR-145 migrated to osteoclasts through extracellular vesicle transport and that these miRNAs triggered osteoclast activity by targeting Cd226 and Srgap2 [108] (Table 3) (Fig. 2).
An increase in TNF-
The overexpression of miR-145 contributes to the activation of the NF-
The anti-inflammatory role of miR-145 was demonstrated by Li et al. (2018) [77]. The authors found that in human adipose tissue, the expression of ADP ribosylation factor 6 (Arf6) is increased and that the amount of miR-145 is reduced in patients with obesity and type 2 diabetes. Studies in the THP-1 cell line have shown that miR-145 inhibits the secretion of proinflammatory factors by reducing Arf6 expression. He et al. (2020) [124] showed that miR-145-based therapy was associated with a significant reduction in inflammatory status. Accordingly, the overexpression of miR-145 reduces inflammation by targeting the OPG and Kruppel-like factor 5 (KLF5) genes.
miR-222 is a component of the miR221/222 cluster, whose members are located on the X chromosome (XP113) [125]. An increase in miR-222 levels has been associated with inflammation [126, 127].
Thus, an increase in blood serum miR-222 levels was positively correlated with the production of proinflammatory cytokines and the concentration of high-sensitivity CRP in patients with calcified aortic valves [126].
Significantly increased levels of miR-221-3p in the synovial membrane of
patients with rheumatoid arthritis and osteoarthritis lead to a decrease in the
anti-inflammatory response by directly targeting the Janus kinase 3
(JAK3) gene, transforming M2 macrophages to the pro-inflammatory M1
type [128]. In a mouse model of rheumatoid arthritis, the TNF-
The aforementioned microRNAs (miR-338-3p, miR-483-5p, miR-133a, miR-151a-3p and miR-125a-5p) can enhance osteoresorption, leading to the disruption of bone tissue homeostasis by inhibiting osteogenesis through various molecular mechanisms (Fig. 2). The roles of miR-128 and miR-145 in regulating osteoclastogenesis are unclear and require further research.
The long-term use of drugs for the treatment of osteoporosis is challenging because of a number of limitations, including severe gastrointestinal side effects, thromboembolism, and increased risk of sarcoma [130]. In this regard, new and safe therapeutic approaches are needed to correct this pathology. MicroRNAs are antagonists of gene expression and can be used to treat osteoporosis and osteoporotic fractures [130]. Notably, existing drugs for the treatment of osteoporosis (e.g., teriparatide and denosumab) may alter the microRNA expression profile. For example, in women with osteoporosis, teriparatide decreased the expression levels of miR-133a and miR-33, and after treatment with denosumab, increases in the expression levels of miR-503 and miR-222 as well as decreases in the activity of the genes related to osteoclastogenesis, namely, RANK and cathepsin K (CTSK), were observed [131].
In the classical approach, microRNAs are used to restore the expression of a missing microRNA. This outcome is often achieved through the use of expression constructs delivered through viral vectors [132].
On the other hand, gene expression can be controlled in other ways. Certain approaches inhibit pathological microRNA expression. The loss of miRNA function can be achieved by using microRNA sponges, microRNA masks, and anti-microRNA oligonucleotides (anti-miRs) [130].
Different types of chemical modifications are used to introduce anti-miR effects [130]. For example, miR-31, miR-106a, and miR-148a inhibit MSC-BM differentiation in osteoblasts by directly targeting RUNX2 [133] (Table 1). After in vitro transfection of anti-microRNA into cells, osteogenic gene expression and ALP activity were increased [133]. Chorionic cells and placental cells were used as alternative sources of MSCs in this study [134]. In addition, inhibition of the expression of specific microRNAs increased the ability of MSCs to undergo osteogenic differentiation culture in vitro, and this strategy can be used to stimulate bone regeneration.
Targeting osteoporosis marker genes for the development of microRNA-based therapies seems to be a promising clinical direction. In addition, curbing excessive osteoclastogenesis can be achieved through the search for molecular switches that direct BMSC differentiation from favoring adipogenesis to favoring osteogenesis [130]. However, no microRNA-based therapy that can be successfully used in the clinic has been found. We analyzed the literature to identify microRNAs with high potential for use as diagnostic markers of osteoporosis, which may also serve as a basis for the development of oligonucleotide therapeutic approaches in the future (Table 4, Ref. [13, 78, 87, 104, 106, 119, 121, 135, 136, 137, 138, 139, 140, 141, 142, 143, 144, 145, 146, 147, 148, 149, 150, 151, 152, 153]).
№ | miRNAs | Material studied | Diagnosis | References |
Decreased microRNA levels | ||||
1 | miR-30b-5b, miR-103-3p, miR-142-3p, miR-328-3p | serum | osteoporosis in postmenopausal women, osteopenia, osteoporosis | [135] |
2 | miR-27 | serum | osteoporosis in postmenopausal women | [136] |
3 | miR-491-5p, miR-485-3p | plasma | osteoporosis in postmenopausal women, vertebral fractures | [137] |
4 | miR-328-3p, miR-22-3p, let-7g-5p | serum | osteoporosis in postmenopausal women, fractures | [138] |
5 | miR-29a-3p, miR-23a-3p, miR-21-5p | serum | [139] | |
6 | miR-144-3p | serum | [140] | |
7 | miR-21-5p, miR-122-5p, miR-125b-5p | serum | osteoporosis, acute hip fractures | [141] |
8 | miR-181c-5p, miR-497-5p | serum | osteoporosis in postmenopausal women | [142] |
9 | miR-550а-3р, miR-532-5р, miR-378а-5р | serum | secondary osteoporosis (idiopathic osteoporosis, osteoporosis in postmenopausal women), fractures | [87] |
miR-365а-3р, miR-324-3р, miR-215-5р, miR-186-5р | ||||
miR-140-5р, miR-93-5р, miR-30е-5р, miR-29b-3p | ||||
miR-19a-3p, miR-19b-3p, miR-16-5p, miR-7-5p | ||||
let-7b-5p | ||||
10 | miR-4516, miR-122-5p | serum | secondary osteoporosis, osteopenia | [78] |
11 | miR-203 | serum | osteoporosis | [143, 144] |
12 | miR-17, miR-20a, miR-21, miR-29a | serum | [145] | |
13 | miR-19a-3p | serum | [146] | |
14 | miR-122-5p, miR-4516 | serum | [78] | |
15 | miR-122-5p | serum | osteoporosis in postmenopausal women | [147] |
Elevated microRNA levels | ||||
1 | miR-133a | serum | osteoporosis in postmenopausal women | [136] |
2 | miR-223-3p, miR-148a-3p, miR-125b-5b, miR-124-3p | serum | osteoporosis | [119] |
miR-122-5p, miR-100-5p, miR-27a-3p, miR-25-3p | ||||
miR-24-3p, miR-23-3p, miR-21-5p | ||||
3 | miR-148a-3p, miR-125b-5p, miR-124-3p, miR-122-5p | serum | osteoporosis in postmenopausal women, fractures | [106] |
miR-100-5p, miR-93-5p, miR-24-3p, miR-23a-3p, miR-21-5p | ||||
4 | miR-125b-5p, miR-122-5p, miR-21-5p | serum | [121] | |
5 | miR-125, miR-30, miR-5914 | serum | [148] | |
6 | miR-125 | serum | [119] | |
7 | miR-2861, miR-124-3p | serum | [139] | |
8 | miR-145, miR-125b, miR-122a, miR-100, miR-27a-3p | serum | [140] | |
miR-24-3p | ||||
9 | miR-133a | serum | [104] | |
10 | miR-532-3p, miR-375, miR-335-5p, miR-152-3p | serum | fractures, low bone mineral density, patients receiving treatment for osteoporosis | [149] |
miR-133b, miR-127-3p, miR-23a-3p, miR-21-5p | ||||
miR-19b-3p | ||||
11 | miR-23b-3p, miR-140-3p | serum | osteoporosis, acute hip fractures | [141] |
12 | miR-148a | serum | osteoporosis in postmenopausal women | [147] |
13 | miR-335-5р, miR-320а, miR-152-3р | serum | secondary osteoporosis (idiopathic osteoporosis, osteoporosis in postmenopausal women), fractures | [87] |
14 | miR-579-3p | serum | osteoporosis | [13] |
15 | miR-410 | serum | osteoporosis in postmenopausal women | [150] |
16 | miR-100 | plasma | osteoporosis | [151] |
17 | miR-375 | serum | [152] | |
18 | miR-208a-3p, miR-155-5p, miR-637 | serum | [153] |
Clinical trials for the use of microRNAs have been performed to study osteoporosis in women [154], men [155], hyperparathyroid patients with and without osteoporosis, and osteoporosis patients undergoing thyroidectomy [156]. MicroRNAs have been studied as potential biomarkers of coronary artery calcification (CAC), and their relationships with bone mineral density and use as markers of bone metabolism have been investigated [157]. Biomarkers (including microRNAs) in orthopedic and maxillofacial surgery patients have been sought to identify risks associated with bisphosphonate use [158]. A study on the relationships between the gut microbiota and bone microarchitecture has also been conducted [159].
In addition, a study on bone-specific microRNAs in the blood serum of postmenopausal women with osteoporosis against a background of antiresorptive or osteoanabolic therapy has been performed. The results are expected to be reported after the end of the study in 2022 [160].
A number of clinical trials have been performed to test oligonucleotide therapies, including treatment with antisense oligonucleotides [161, 162, 163, 164].
However, despite the many approaches used to stabilize oligonucleotides in cells, oligonucleotide bioavailability is still low, and their efficient delivery to target organs other than the liver remains a challenge [165]. Increasing the concentration of administered oligonucleotides can lead to toxic side effects [166]. Therefore, methods for the delivery of oligonucleotides via exosomes are under development for future application [167].
Osteoporosis remains an incurable and serious multifactorial disease. The relationship between osteoporosis and obesity and the regulation of osteoclast activity, in particular, is detailed in a review by Roy et al. (2016) [9]. Recently discovered miRNAs involved in the epigenetic control of mRNA play important roles in regulating the adipogenic/osteogenic differentiation of BMSCs and osteoclastogenesis [168, 169, 170].
In the present review, we summarize recent findings on the regulatory interactions between mRNAs and miRNAs in the regulation of BMSC differentiation and the activation of osteoclastogenesis in obesity and osteoporosis. We hypothesize that miR-122, miR-21, miR-125, miR-23-3p, miR-140, miR-2861, and miR-133 have high potential for use in the diagnosis of osteoporosis. However, in view of the large body of work devoted to the search for biomarkers of pathologies, this finding remains to be confirmed because the changes in miRNA levels in blood serum/plasma have multidirectional dynamics.
Many studies show the promising possibility of using short-stranded noncoding RNAs for therapeutic purposes [171, 172]. The complex regulation of the expression of genes important for the balance between osteogenesis and osteolysis gives us hope for the development of a safe therapy for osteoporosis. To reduce the severity of osteoporosis, treatment should ideally target the following: the hypoactivation of osteoclasts, the restoration of osteoblast function, the prevention of adipogenic differentiation in BMSCs, and the restoration of bone perfusion. In this regard, to reduce the severity of adipogenesis in BMSCs, we suggest therapy with inhibitors, such as miR-204, miR-211, miR-217, miR-221, miR-222, miR-128, miR-140, miR-16, miR-188, miR-143, miR-145, and miR-532. The positive regulation of osteoblastogenesis in BMSCs can be achieved by increasing the levels of miR-296-3p, miR-2861, miR-3960, miR-335, and miR-29a mimetics in the BMSC microenvironment.
Perhaps, to inhibit osteoclast hyperactivation, therapy with miR-483 inhibitors or miR-338, miR-133a, miR-151, and miR-125 mimetics should be considered. The ambiguous roles of miR-128 and miR-145 in the regulation of osteoclasts require further research.
The relevance of the search for targeted miRNAs stems from the fact that promoting the reparative regeneration of bone tissue (osteosynthesis) under its pathological remodeling conditions in osteoporosis is an unsolved clinical problem. Among the numerous available drugs, BMPs have direct bone tissue regenerative properties [173]. However, when administered systemically, they cause numerous side effects (including bone resorption) in 20–70% of cases, which negates the benefit of their specific osteogenic activity [174]. An obstacle to the widespread use of BMPs is also their high price (due to high costs in the research and development phase), which is disproportionate to the therapeutic efficacy of the drugs.
In this context, the therapeutic use of miRNAs, which modulate the (post)transcriptional activity of genes at the mRNA level, is considered a promising new biotechnological direction in regenerative medicine. However, a number of unresolved issues remain, especially regarding the potential side effects caused by (multiple) crossover effects of microRNAs on intracellular signaling pathways, which may lead to unpredictable responses in cells of nontarget tissues and organs in the long term.
Therefore, the development of local systems for the targeted delivery and release of microRNAs (capsules of different sizes, nanoparticles, scaffolds, etc.) that overcome tissue barriers independently [175] or with the help of carrier cells (e.g., MSCs) [176] is an urgent task to be addressed in the near future.
BMSCs, bone marrow mesenchymal stem cells; MSCs, mesenchymal stem cells; BMI,
body mass index; TNF, tumor necrosis factor; IL, interleukin; UTRs, untranslated
regions; RANKL, receptor activator of nuclear factor kappa-B ligand; WNT,
Wingless-type MMTV integration site; BMP, bone morphogenic protein; RUNX,
runt-related transcription factor; NF-kB, nuclear factor-
MV, NT, KY (Author A, C and D)—original draft preparation; IKh, LL, IK (Author B, F and J)—editing; MV, NT, KY, VM, DS, AK, OKh (Author A, B, C, D, E, G, H)—gathered information and performed material systematization; MV (Author A) visualization. All authors contributed to editorial changes in the manuscript. All authors read and approved the final manuscript.
Not applicable.
Not applicable.
This research was funded by the State Assignment Grant number FZWM-2020-0010, the Grant of the President of the Russian Federation number MK-2072.2022.3 and Immanuel Kant Baltic Federal University and Siberian State Medical University Development Program Priority 2030.
The authors declare no conflict of interest.