- Academic Editors
Background: Nasopharyngeal carcinoma (NPC) is a malignant tumor
associated with Epstein-Barr virus (EBV) infection. Chemoradiotherapy is the
mainstream treatment for locally advanced NPC, and chemotherapeutic drugs are an
indispensable part of NPC treatment. However, the toxic side-effects of
chemotherapy drugs limit their therapeutic value, and new chemotherapy drugs are
urgently needed for NPC. Silvestrol, an emerging natural plant anticancer
molecule, has shown promising antitumor activity in breast cancer, melanoma,
liver cancer, and other tumor types by promoting apoptosis in cancer cells to a
greater extent than in normal cells. However, the effects of silvestrol on NPC
and its possible molecular mechanisms have yet to be fully explored.
Methods: Cell counting kit-8 (CCK-8), cell scratch, flow cytometry,
5-ethynyl-2
Nasopharyngeal carcinoma (NPC) is a malignant tumor that develops in the upper part of the throat behind the nose. It has a distinct geographical distribution and is more common in East and Southeast Asia. The incidence of NPC in China ranges from 15 to 50 cases per 100,000 population and shows a decreasing trend from south to north due to environmental and genetic factors [1, 2, 3]. The incidence is slightly higher in males than females [4, 5], and individuals with a positive family history have a four-fold increased risk of developing NPC [6].
The incidence of NPC is associated with Epstein-Barr virus (EBV) infection [7].
However, as of my last update, vaccines against EBV infection have shown
potential but have not been widely implemented or shown to significantly reduce
the incidence of NPC. Over the past decade, radiotherapy and chemoradiotherapy
have been the main treatments for NPC. Radiotherapy is the primary treatment for
early-stage NPC as it is highly sensitive to ionizing radiation. However,
radiotherapy alone has limited efficacy in T2N1 patients, and early treatment of
these patients should be combined with chemotherapy [8]. For stage I-II NPC,
intensity-modulated radiation therapy (IMRT) with or without chemotherapy has
achieved a 5-year survival rate of
Silvestrol, a natural plant-derived anticancer molecule, has shown promising antitumor activity in breast cancer [15], melanoma [16], liver cancer [17], and other tumor types. It promotes a greater extent of apoptosis in cancer cells compared to normal cells [18]. Singh et al. [19] demonstrated that silvestrol binds specifically to eIF4A and selectively inhibits the translation of GQ-enriched messenger RNAs (mRNAs) such as KRAS and its downstream signaling molecules. eIF4A plays a role in various cellular processes, and silvestrol exhibits varying effectiveness in different cancer types. This study aims to uncover the specific anti-tumoral effects and molecular mechanisms of silvestrol in NPC cells, with the ultimate goal of discovering new treatments for NPC patients.
The human NPC cell lines HONE1 (Mingzhoubio, MZ-1647, Ningbo, China) and CNE2
(Immocell, IM-H136, Xiamen, China) were cultured in DMEM (Procell,
PM150210, Wuhan, China) with 10% fetal bovine serum (Procell,
164210-500, Wuhan, China) and 1% penicillin-streptomycin double antibiotic
(Beyotime, C0222, Shanghai, China) in a cell incubator at 37
°C and 5% CO
BeyoClickTM EdU-488 (Beyotime, C0071S, Shanghai, China) was used for the
proliferation assay. HONE1 and CNE2 cells were seeded in 96-well plates (5
Cells were cultured for 24 h until 90% confluence was achieved. The monolayer
was scratched with the tip of a pipet gun and the exfoliated cells removed. Cells
were then cultured in serum-free medium containing silvestrol with or without
LY3214996 (Medchemexpress, HY-101494, Monmouth Junction, NJ, USA). The cell migration rate was
calculated as follows: (Initial Scratch Width – Scratch Width after 24
h)/Initial Scratch Width
A total of 1.5
Silvestrol with or without LY3214996 was added to the medium of the experimental group. Trypsin digestion was carried out to harvest cells, followed by PBS washing and subsequent fixation in 75% alcohol for 24 h. Staining procedures were performed with PI/RNase Staining Buffer (BD Biosciences, 550825, San Diego, CA, USA).
Cells were lysed in RIPA lysis buffer (Beyotime, P0013J, Shanghai, China) and
1% PMSF (Solarbio, P0100, Beijing, China) and then centrifugated. The extracted
proteins were denatured with SDS-PAGE protein loading buffer (Beyotime,
P0015, Shanghai, China) (100 °C, 10 min) and separated by 11% SDS-PAGE gel electrophoresis
(Beyotime, P0903, Shanghai, China). Proteins were then transferred onto PVDF
membranes (Beyotime, FFP22, Shanghai, China) and blocked with a
5% BSA (Beyotime, ST023, Shanghai, China) solution. Incubation with primary
antibodies was carried out overnight at 4 °C, followed by subsequent incubation
with horseradish peroxidase-labeled secondary antibodies for 1.5 h. The
ultra-sensitive multifunctional imager (Cytiva, Amersham ImageQuant 800,
Shanghai, China) was used to visualize protein bands. The following antibodies
(Affinity, Wuhan, China) were used:
The age range of the patients providing the pathological tissue sections is 50-60 years old. The providers of tissues #1 and #3 are male, while the provider of tissue #2 is female. Tissue sections (4 µm) (Collection date: #1 - June 2022, #2 - November
2022, #3 - May 2022) were incubated at 60 °C for 20 minutes before undergoing
xylene (Biofount, 1330-20-7, Beijing, China) deparaffinization
and ethanol rehydration. Antigen retrieval was performed in 10 mM citric acid
solution (Beyotime, P0081, Shanghai, China) and involved microwave pretreatment
(15 min) and subsequent cooling. Endogenous peroxidase activity was quenched with
H
CNE2 and HONE1 cells were separated into two experimental groups: silvestrol (20
nM), and silvestrol (20 nM) + LY3214996 (80 nM). Cell samples were frozen in
TRIzol reagent (Solarbio, 15596-018, Beijing, China) and submitted to Geneplus
Technology Corporation (Shenzhen, China) for RNA sequencing. Any mRNA with a
fold-change
Prism (GraphPad Software, GraphPad 8.0.1, San Diego, CA, USA) was used to compare groups.
All data were expressed as the mean
Flow cytometry revealed that 20 nM silvestrol significantly increased the
apoptosis rate in HONE1 and CNE2 NPC cells (*p
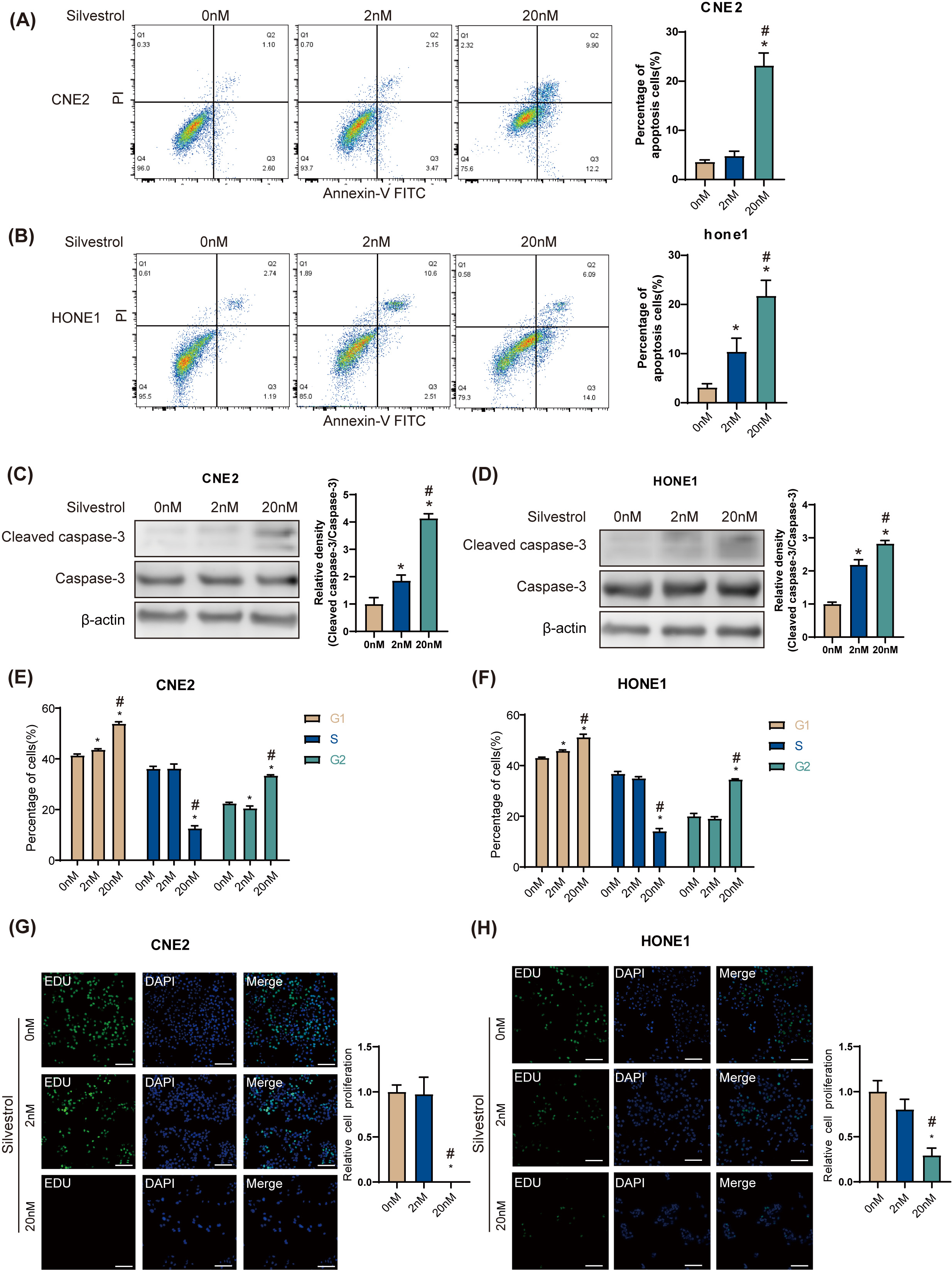
Silvestrol promotes apoptosis and inhibits proliferation of
nasopharyngeal carcinoma (NPC) cells. NPC cells were treated with silvestrol at
different concentrations (0 nM, 2 nM, 20 nM) for 24 h. (A,B) Apoptosis was
determined by flow cytometry in CNE2 and HONE1. (C,D) Western blot (WB)
determined cleaved caspase-3 expression in CNE2 and HONE cells. (E,F) Cell cycle
was determined by flow cytometry in CNE2 and HONE1. (G,H) Cell proliferation was
measured by 5-ethynyl-2
The results of the Western Blot (WB) assay showed that p-ERK and p-MEK protein
levels were significantly increased in CNE2 and HONE1 cells between 0.5 h and 2 h
after silvestrol treatment (*p
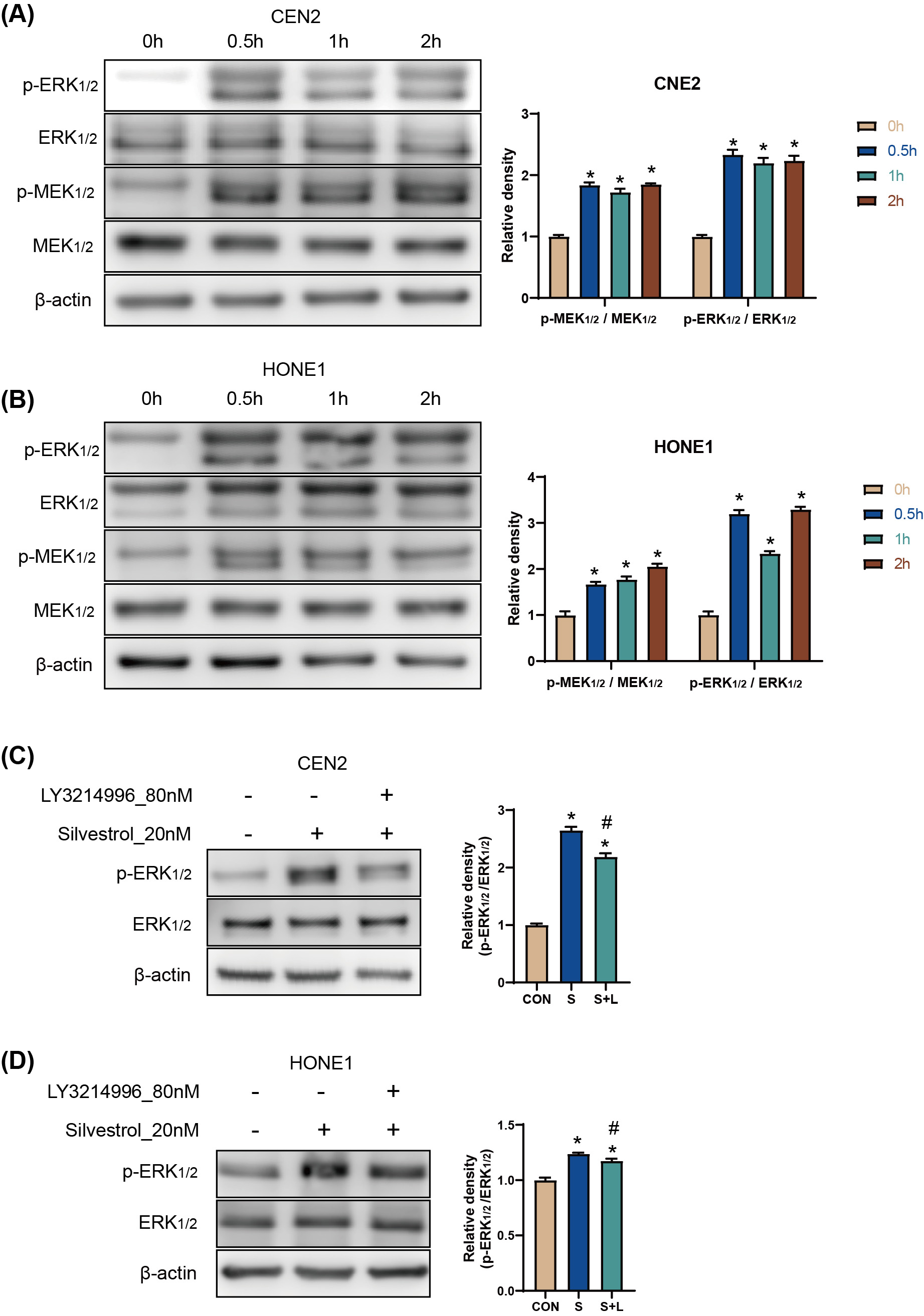
Silvestrol activates the ERK pathway by promoting ERK
phosphorylation. (A,B) WB detection for p-ERK/ERK, andp-MEK/MEK protein
expression in CNE2 and HONE1 cells. Silvestrol for 0 h, 0.5 h, 1 h and 2 h. The
values are performed as mean
Treatment with 20 nM silvestrol increased the apoptosis rate of HONE1 and CNE2
cells significantly (*p
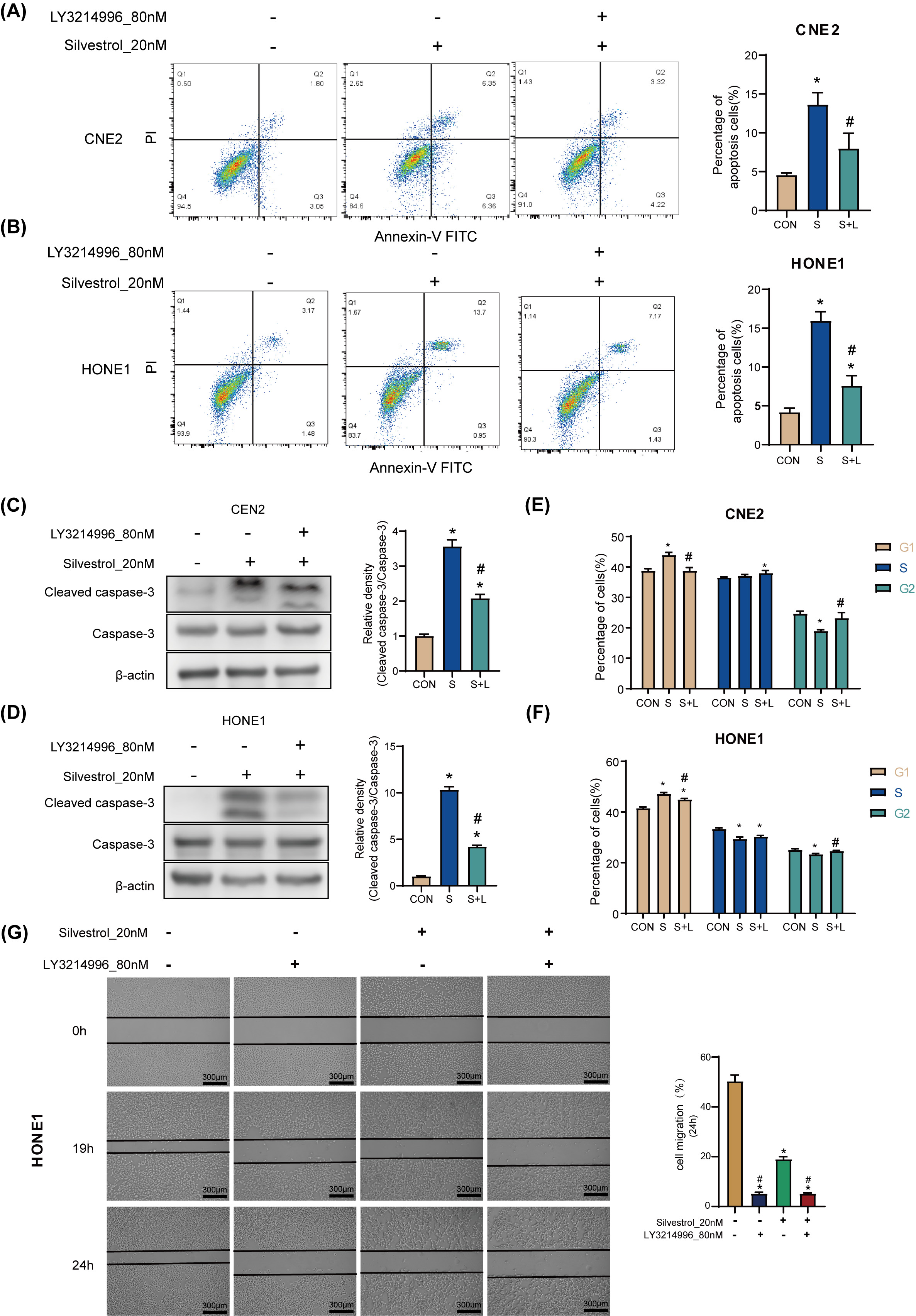
Silvestrol acts on NPC cells through the ERK pathway.
(A,B) Apoptosis was determined by flow cytometry in CNE2 and HONE1. (C,D) WB
detection for cleaved caspase-3 expression in CNE2 and HONE cells. (E,F) Cell
cycle was determined by flow cytometry in CNE2 and HONE1. (G) Cell migrations of
HONE1 in response to different drug treatments. NPC cells were treated with
silvestrol and/or LY3214996 for 1h (CON, control group without drug; S,
single-drug group with 20 nM silvestrol; S + L, two-drug group with 20 nM
silvestrol and 80 nM LY3214996), Bar = 300 µm; The values are performed as
mean
A total of 960 DEGs, related to both CNE2 and HONE1 cells as well as broader NPC research available in databases like the Comparative Toxicogenomics Database (CTD) (http://ctdbase.org/), were identified through Venn diagram analysis (Fig. 4A). Of these, 572 hub DEGs displayed consistent expression trends across CNE2 and HONE1 cells, comprising 303 down-regulated genes and 269 up-regulated genes. Protein network analysis of the up-regulated genes highlighted two protein interaction networks, with RAP1A protein identified as a core element in both (Fig. 4B,C). Furthermore, the tight junction pathway, involving RAP1A, emerged as the top-scoring pathway in the KEGG pathway analysis, indicating significant changes in pathways associated with the up-regulated DEGs (Fig. 4D). For the down-regulated genes, hexokinase-II (HK2), PFKM, PGAM1, and PKM proteins were all pivotal members of their respective protein interaction network (Fig. 4E). These proteins are integral to carbon metabolism and glycolysis/gluconeogenesis pathways (Fig. 4G), which were highlighted as the top three pathways in KEGG pathway enrichment analysis (Fig. 4F).
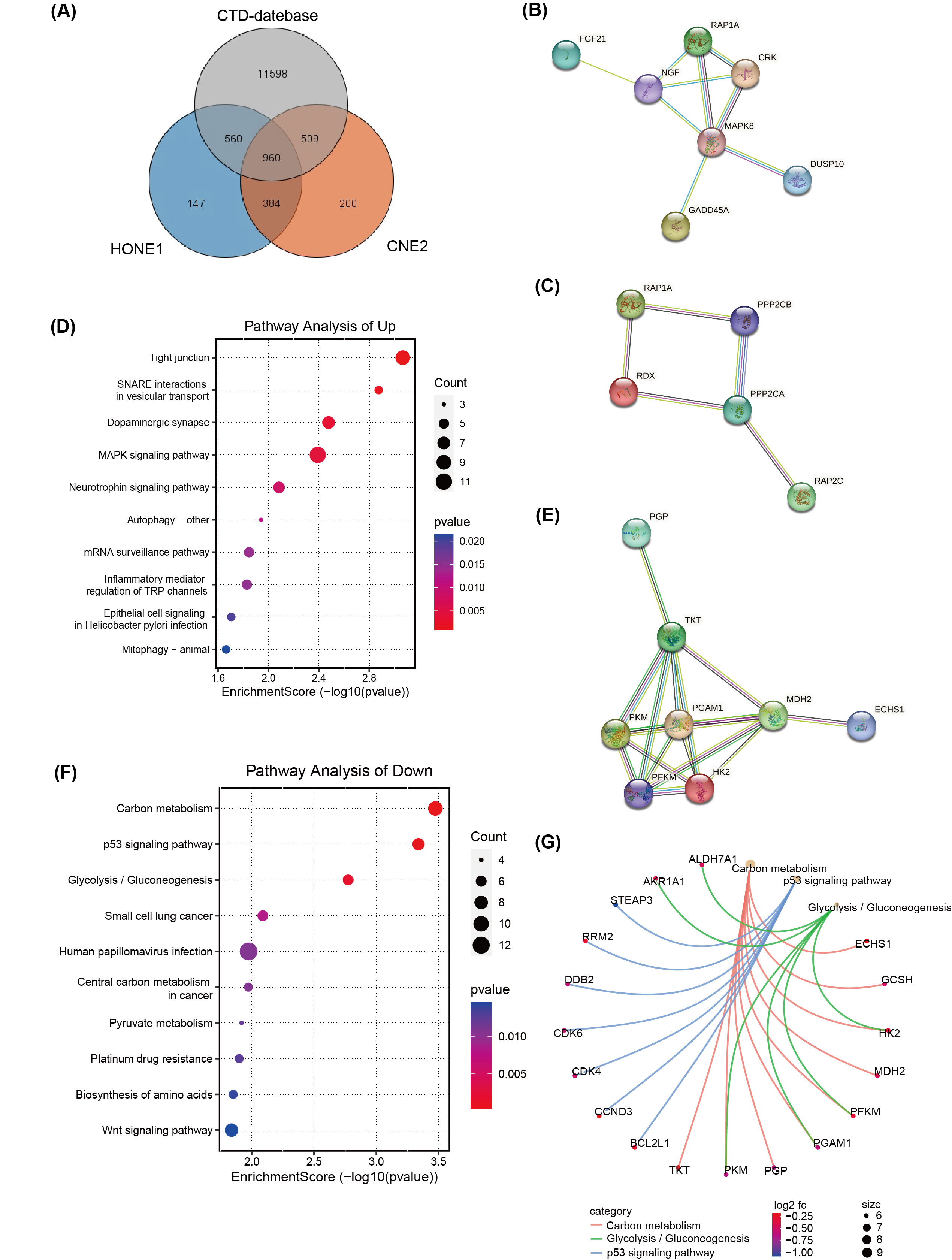
Transcriptome differences in NPC cells treated with
silvestrol and with or without ERK inhibitors. (A) Venn diagram of Comparative
Toxicogenomics Database (CTD) database, TCGA and RNA-seq. (B,C) The
protein-protein interaction (PPI) network of up-regulated hub DEGs
(
Treatment with 20 nM silvestrol significantly decreased RAP1A, HK2, and GADD45A
protein expression in CNE2 and HONE1 cells compared to the control group
(*p
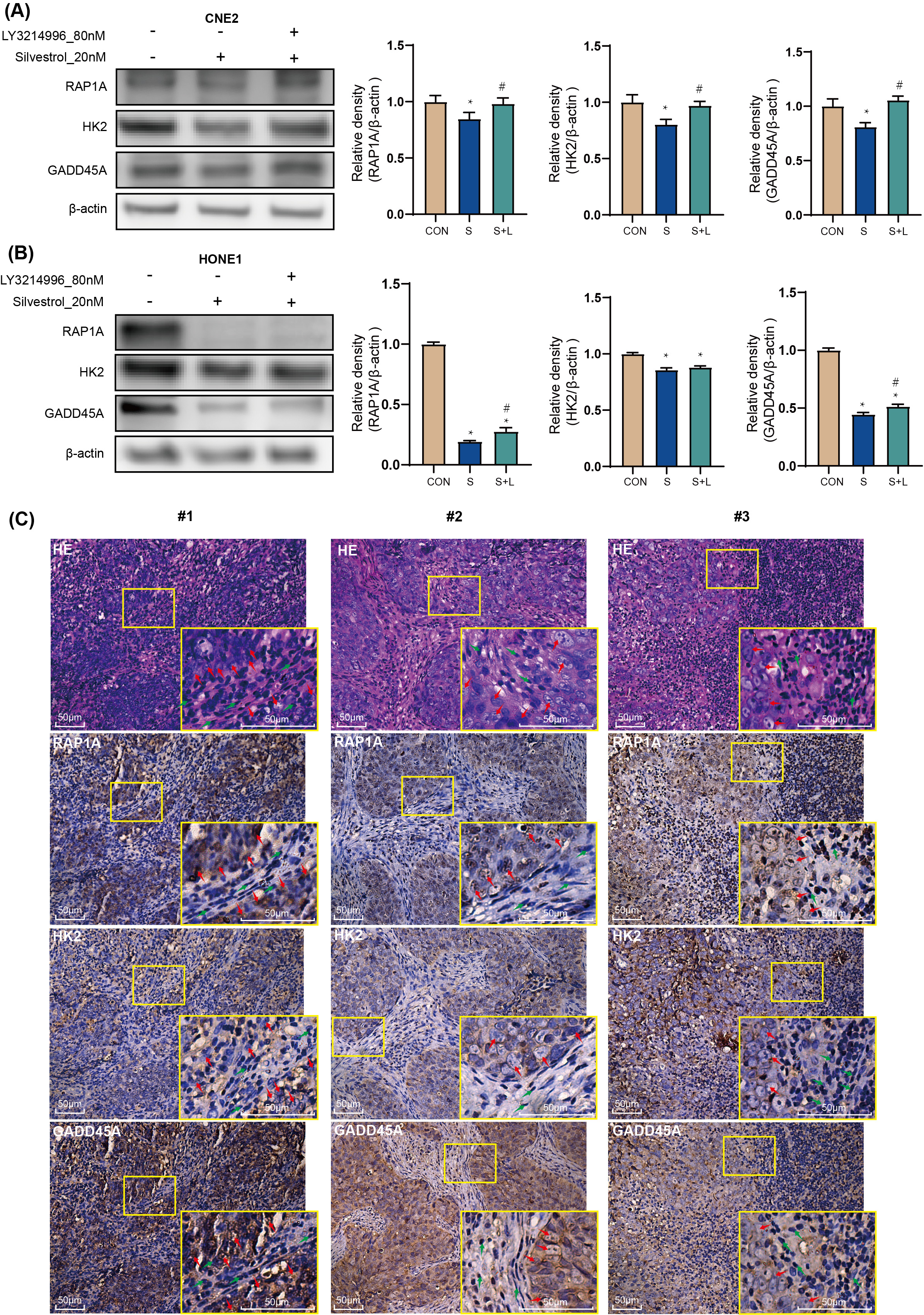
Target genes: the effect of silvestrol on protein
expression in NPC patient tissue. NPC cells were treated with silvestrol with or
without LY3214996 for 1 h (CON, control group; S, 20 nM silvestrol; S + L, 20 nM
silvestrol and 80 nM LY3214996). The expression level of GADD45A, RAP1A and HK2
proteins was evaluated by WB assay in (A) CNE2 cells, and (B) HONE1 cells. (C)
H&E tissue sections from NPC patients, and IHC of GADD45A, RAP1A and HK2. Red
arrows, tumor cells; Green arrow, non-tumor cells. Bar = 50 µm. The values
are performed as mean
The specific location of NPC results in a relatively high risk of side effects from radiation therapy. This study investigated silvestrol, a novel small-molecule compound, for its potential to inhibit the occurrence and development of NPC. Silvestrol is known to target EIF4A, inhibiting the cellular translation process with some selectivity for mRNA [20, 21]. Our findings demonstrated that silvestrol promotes cell apoptosis, inhibits cell cycle progression, and increases the phosphorylation of ERK and MEK. While previous studies have indicated that downregulation of the MEK/ERK pathway can inhibit NPC cell proliferation, migration, invasion [22, 23], and promote apoptosis [24], it’s important to note that activation of the ERK pathway can both promote cell proliferation and apoptosis, suggesting its role as a double-edged sword [25]. In addition, the phosphorylation of ERK1/2 under the action of GBP3 can promote the proliferation of brain glioma cells [26].
RAP1A, a member of the RAS oncogene family, is implicated in various tumor cell processes including proliferation, invasion, migration, and apoptosis [27, 28, 29, 30]. In addition, a study on long non-coding RNA suggests that RAP1A promotes the metastasis of NPC cells [31]. In our study, RAP1A emerged as a central node in protein interaction networks among up-regulated genes, particularly within the tight junction pathway. Despite the observed inhibition of RAP1A expression by silvestrol, co-treatment with the ERK pathway inhibitor LY3214996 reversed this effect. Immunohistochemistry (IHC) in NPC patient tumor tissue confirmed RAP1A overexpression, linking silvestrol-mediated ERK pathway activation to the regulation of RAP1A expression and its association with NPC cell apoptosis and proliferation.
HK2, a critical enzyme in glycolysis, was identified as a core member of the
network formed by down-regulated genes. HK regulates the metabolic processes of
aerobic glycolysis, autophagy, cell death, and intracellular Ca
GADD45A, associated with growth arrest and DNA damage response [36, 37], also showed differential expression in response to silvestrol and LY3214996. A study of melanoma cells found that the MAPK-ERK pathway regulates GADD45A, and that down-regulation of GADD45A promotes G2/M arrest and apoptosis [38]. Its role in cell cycle checkpoints and apoptosis aligns with our observations on cell cycle arrest and apoptosis induction in NPC cells treated with silvestrol. The variability in GADD45A expression across different patient tissues points to the need for further investigation into its regulation and role in NPC pathogenesis.
RAP1A, HK2, and GADD45A emerge as critical genes through which silvestrol may exert its anti-proliferative effects on NPC cells. However, the specific mechanisms underlying these effects and the potential interplay with the ERK pathway require deeper exploration. Further research into these genes and their regulation is vital not only for elucidating silvestrol’s antitumor mechanism but also for advancing our understanding of NPC carcinogenesis.
Silvestrol was found to affect the expression of RAP1A, HK2, and GADD45A proteins, with its action leading to the inhibition of NPC cell proliferation and the promotion of apoptosis. Specifically, silvestrol’s effects on RAP1A, HK2, and GADD45A expression appear to be mediated through the activation of the ERK pathway. Moreover, silvestrol inhibits cell migration through mechanisms that do not directly involve the ERK pathway, potentially implicating the role of RAP1A in these non-ERK pathway-related effects. This dual mechanism of action highlights silvestrol’s potential as a multifaceted therapeutic agent in the treatment of NPC, targeting both cell proliferation and migration to inhibit cancer progression.
The datasets generated and analyzed during the current study are available in the SRA repository, [https://dataview.ncbi.nlm.nih.gov/object/PRJNA946356?reviewer=a6s5rqba44sf6thtv4tpsitbm0]. Additionally, other datasets used and/or analyzed during the current study are available from the corresponding author on reasonable request.
LY led the methodology, validation, formal analysis, writing - original draft, and data curation. JF, JD, and WY conducted the validation process and collaborated with LY to finalize the writing of the “Materials and Methods” section, as well as ensuring the accuracy of the “Results” section in manuscript. DL, XQ, and XL provided supervision and project administration, were responsible for the overall project management and supervision, and participated in the conceptualization and design of the manuscript, as well as critical review of the key content. XH, DL, and YT were responsible for Writing - review and editing and Visualization; they conducted significant critical reviews and editing of the manuscript content. All authors read and approved the final manuscript. All authors have participated sufficiently in the work and agreed to be accountable for all aspects of the work.
This study was conducted in accordance with the Declaration of Helsinki and approved by the Ethics Committee of Chongqing General Hospital (protocol code: KY S2022-073-01, date: 2022.03.11). Written informed consent was obtained from all participants before entering the study.
We would like to express our profound gratitude to BETTER BIOTECH Research Institute for providing the research platform for the immunohistochemistry (IHC), Western Blot (WB), and cell culture experiments conducted in this paper.
This research received no external funding.
The authors declare no conflict of interest.
Publisher’s Note: IMR Press stays neutral with regard to jurisdictional claims in published maps and institutional affiliations.