- Academic Editor
†These authors contributed equally.
‡These authors contributed equally.
Background: The infection and negative effects of the SARS-CoV-2
(severe acute respiratory syndrome coronavirus) virus are mitigated by vaccines.
It is unknown whether vaccination has worked by eliciting robust protective
innate immune responses with high affinity. Methods: Twenty healthy
volunteers received three doses of Comirnaty (Pfizer Australia Pty Ltd.) and were
evaluated 9 months after the second vaccination and 1 month after the booster
dose. The exclusion criteria were the presence of adverse effects following the
vaccination, a history of smoking, and heterologous immunization. The inclusion
criteria were the absence of prior Coronavirus Disease (COVID)-19 history, the
absence of adverse effects, and the absence of comorbidities. Specific phenotype
and levels of CD107a and granzyme production by blood NK (natural killer) cells
were analyzed after exposure to SARS-CoV-2 spike antigen (Wuhan, Alpha B.1.1.7,
Delta B.1.617.2, and Omicron B1.1.529 variants), and related with anti-SARS-CoV-2
antibody production. Results: The booster dose caused early NK CD56
The severe acute respiratory syndrome coronavirus, sometimes known as SARS-CoV-2 (severe acute respiratory syndrome coronavirus), is vaccine-preventable [1]. High affinity and persistent protective antibody responses indicate an efficient humoral immune response to vaccination [2]. By six months, there is a significant reduction in antibody responses, especially after vaccinations against SARS-CoV-2 mRNA [3].
A recent investigation found that the protection against Coronavirus Disease (COVID)-19-related hospitalization and death started to decrease in older adults and patients with weak or numerous medical conditions after 20 weeks [4]. Natural killer (NK) cells are essential for antiviral immunity. Virally infected host cells can be killed by NK cells by triggering apoptosis in various ways. Two of the proteins that it can first exocytose are perforin and granzymes, and when they interact, they can cause the target cell to undergo apoptosis [5]. By expressing the executioner molecules Fas cell surface death receptor (FAS) ligand (FasL) [6] and Tumor Necrosis Factor (TNF)-related apoptosis-inducing ligand (TRAIL) [7, 8], NK cells can destroy the target. This stimulates the signaling of the extrinsic apoptotic pathway. NK cells can also release chemokines and pro-inflammatory cytokines such as Interferon (IFN)-gamma, TNF-beta, and granulocyte-macrophage colony-stimulating factor (GM-CSF) [9]. By encouraging pro- or anti-inflammatory tendencies as a disease progresses, NK cells exhibit functional plasticity [9].
Peripheral blood contains about 10–15% of human NK cells, which are recognized
phenotypically by the presence of CD56 and CD16 on their surfaces and the absence
of CD3. Based on the surface expression of CD56 and CD16, two subgroups of NK
cells have been discovered in humans: CD56(+)
Increased evidence suggests that the SARS-CoV-2 infection may affect the tissue
distribution and effector capabilities of NK cells and that a rapid NK cell
response may determine a patient’s clinical outcome. However, more research is
required to pinpoint the precise role of NK cells in the pathophysiology of
COVID-19. Patients who have SARS-CoV-2 infection experience lymphopenia as a
symptom. In patients with severe infections, neutrophils and monocytopenia
frequently coexist with this lymphopenia [11]. Numerous independent studies
revealed that the amount of NK cells in the bloodstream is also influenced by the
SARS-CoV-2 infection [12, 13] without differences in the location of NK cell
subsets. This decrease in circulating NK cells appears to be related to the
illness’s severity and the acute stage [14, 15]. NK cell counts gradually
decrease in patients with a fatal course of the illness following the onset of
symptoms, contrary to what has been demonstrated for T and NK cell numbers, which
are increased in the latter stages of the illness [12, 13, 16]. According to
recent studies, the quantity of NK cells in hospitalized patients and the rate of
viral load reduction are directly related. Circulating NK cell counts may be used
as a prognostic clinical parameter to predict the course of COVID-19. Patients
with “normal” (
In this study, we tracked the development of circulating NK cells after Comirnaty (Pfizer Australia Pty Ltd) vaccination, concentrating on the activation of NK cells, given their relevant role in infection control.
Twenty healthy volunteers were enlisted for the study. The exclusion criteria were the presence of adverse effects following the vaccination, a history of smoking, and heterologous immunization. The inclusion criteria were the absence of prior COVID-19 infection, the absence of adverse effects, and the absence of comorbidities. Following approval by the Area Vasta Emilia Centro della Regione Emilia-Romagna (CE-AVEC) ethics committee (protocol # 122/2021/Oss/AOUFe), samples were obtained with informed consent. Table 1 lists the demographic characteristics.
Female, n (%) | 50 (10) |
Age (years), mean (SD) | 36 (10) |
Smoker, n (%) | 0 (0) |
Comorbidities n (%) | 0 (0) |
mRNA vaccine* | 20 (100%) |
Blood sample after second immunization (months |
9.0 |
Blood sample after booster dose (months |
1.0 |
*All the subjects received three doses of Comirnaty (Pfizer Australia Pty Ltd.).
Peripheral blood mononuclear cells (PBMCs) collected 9 months after the second vaccination and 1 month after the booster dose with mRNA vaccines were isolated from the whole blood of healthy donors by centrifugation using Lymphocyte separation medium (LSM; Corning; Merck Life Science S.r.l., Milan, Italy).
LSM (Corning; Merck Life Science S.r.l., Milan, Italy) was used to separate
peripheral blood mononuclear cells (PBMCs) from whole blood of healthy donors 9
months after the second vaccination and 1 month after the booster dose with mRNA
vaccines. Cells were plated at a density of 1
For five days, adherent cells were grown in specific media (CellGro DC, CellGenix; Freiburg, Germany) with 1000 IU/mL of GM-CSF (granulocyte-macrophage colony-stimulating factor) and 50 ng/mL of IL-4 (interleukin-4) (R&D System). Three spike protein antigens (Wuhan, Alpha B.1.1.7, Delta B.1.617.2, and Omicron B1.1.529 full-length spike protein, BioServ, Flemington, NJ, USA) were added to the antigen-presenting cells on day 4 at a concentration of 40 µg/mL for 24 h. Tumor necrosis factor-alpha (20 ng/mL), interleukin-1 (10 ng/mL), and interferon-gamma (1000 IU/mL) were used to stimulate the final antigen following cell maturation for two days [19].
According to product instructions, NK cells were extracted from peripheral blood
samples using the positive magnetic cell separation technique (Miltenyi Biotech,
Gladbach, Germany). According to flow cytometry results using CD56-PerCp-Cy5.5
and CD16-FITC moAbs from e-Bioscience in Frankfurt, DE, the NK cell concentration
was
A total of 10
Autologous pure NK cells were stimulated with spike-loaded antigen-presenting
cells, as reported in Section 2.4. As a positive control, NK cells were treated
with 25 ng/mL PMA (Sigma, St. Louis, MO, USA) and 1 µM ionomycin
(Sigma, St. Louis, MO, USA). NK cells were stained with the following antibodies
(Biolegends): APC mouse anti-human CD3, PerCP-vio700 mouse anti-human CD56, FITC
mouse anti-human CD16, PE mouse anti-human NKG2A, NKG2D, NKG2C, CD127, and CD57.
The samples were incubated for 30 minutes with the moAbs or anti-isotype controls
(Exbio, Praha, CZ) in ice, washed, and analyzed with FACS Aria flow cytometer and
FlowJo software (Becton Dickinson, San Jose, CA, USA), acquiring 10,000 events.
Lymphocytes were identified according to forward/side scatter profile, and NK
cells (CD3
Plasma samples collected from vaccinated control subjects were evaluated by
commercial ELISA assay for anti-RBD (receptor binding domain) IgG levels
(ThermoFisher Scientific, Milan, Italy), following assay protocols. Anti-spike
RBD IgG was considered specific for SARS-CoV-2 spike protein when a ratio
In vitro testing was done to determine the anti-SARS-CoV-2 infection-specific anti-spike antibodies’ neutralizing power [20]. The SARS-CoV-2 inoculum was donated by Prof. Caruso of the University of Brescia in Italy and was isolated from a nasopharyngeal swab taken from a patient with COVID-19 (a Caucasian man of Italian descent, genome sequences available at GenBank (SARS-CoV-2-UNIBS-AP66: ERR4145453). This SARS-CoV-2 isolate belonged to the B1 clade, which also comprises the majority of Italians and sequences from other European and American nations. Plaque assay was used to measure the virus titer in Vero E6 cells, as previously mentioned [12]. Patient plasma samples were used to cultivate Calu-3/SARS-CoV-2-infected cells. The viral load was determined by real-time PCR for the SARS-CoV-2 genome performed on RNA extracted from cell supernatants, compared to infected controls. The neutralization was reported as the decreased viral load percentage.
RNA extraction was performed by using the MagMAX Viral/Pathigen Nucleic Acid Isolation kit (ThermoFisher, Italy) according to the manufacturer’s instructions, 48 h post-infection (hpi) [21]. SARS-CoV-2 titration was performed by RealTime-PCR with the TaqMan 2019nCoV assay kit v1 (ThermoFisher, Italy).
Statistical analysis was performed by a parametric approach for the normal
distribution, as assessed using the Kolmogorov–Smirnov test. Fisher’s exact test
was used to compare percentage frequencies, and Student’s t-test was
used to compare variables. Spearman’s correlation test was used to evaluate
linear regression. A value of p
The sera of healthy donors showed significant spike-binding and neutralizing antibody variability following a second immunization (Fig. 1a,b). We observed an increase in the spike-binding and neutralizing antibody levels 1 month after the booster dose (Fig. 1a,b) (Student’s t-test, Fisher’s exact test, p = 0.001 respectively), demonstrating the success of the booster dosage in enhancing the humoral response towards SARS-CoV-2.
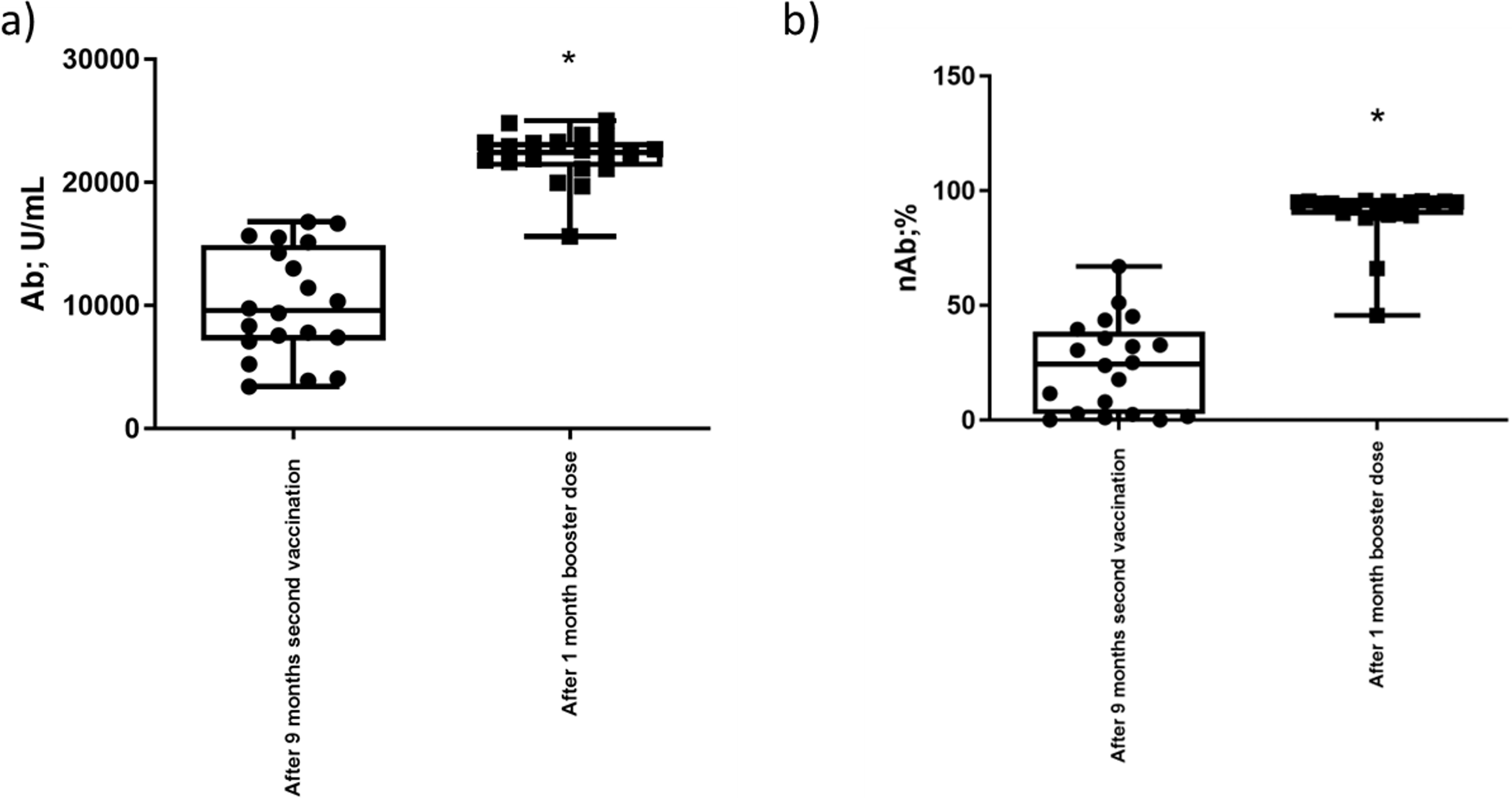
Anti-Spike IgG evaluation. (a) Anti-spike SARS-CoV-2
RBD IgG (Ab) plasma levels in 20 healthy individuals 9 months after the second
vaccination and 1 month after the booster dose. The results are reported as the
mean
We examined the immunological profile of NK cells nine months after the second
vaccination and 1 month after the booster dose since the innate immune response
is an important part of the protective immune response to infections [22, 23].
Between 9 months after the second vaccination and 1 month after the booster dose,
we detected no variation in the frequency of CD3
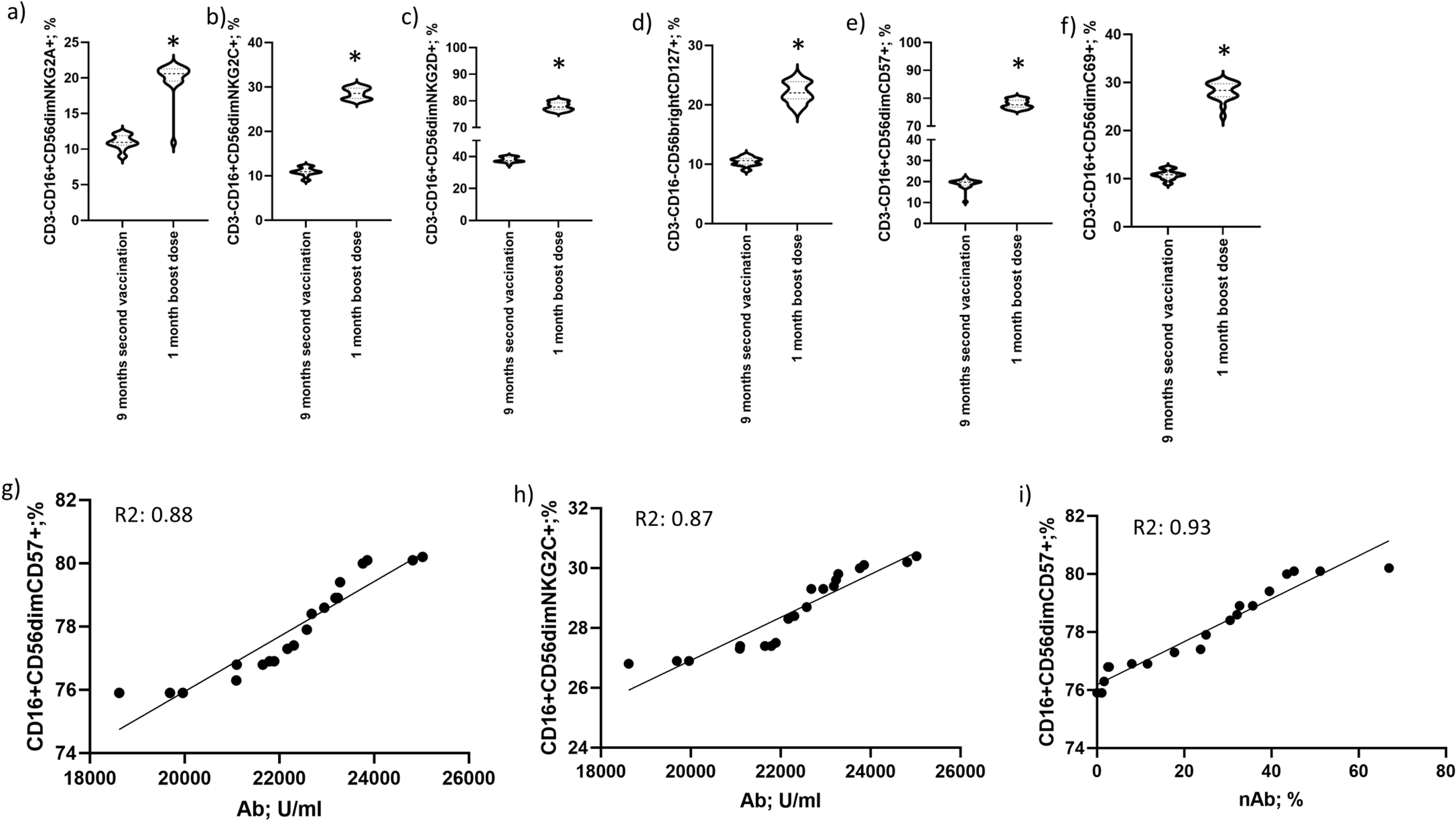
NK cell immunophenotype. Differential profile of
CD56
The results revealed considerable differences in NK surface markers expression 1
month after the booster dose, including a high level of NKG2A/C/D
Since there is evidence that at least some NK-cell subsets, such as the rise in
NKG2C
We determined how frequently NK cells with CD16
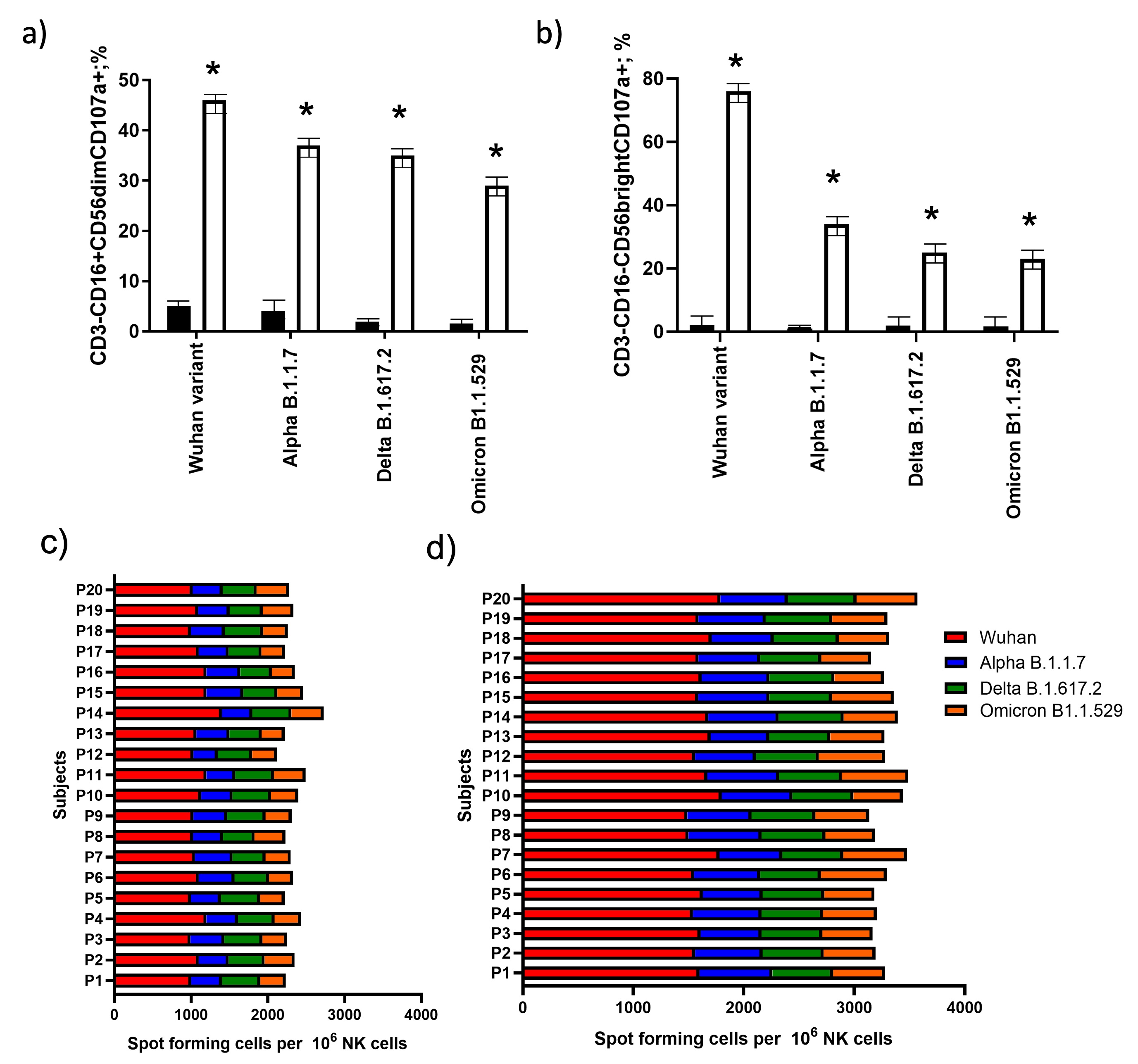
NK cell activation and subset evaluation. The number
of (a) CD56
The 1-month post-boost dose group showed enrichment of both
CD56
The elimination of viruses such as SARS-CoV-2 is known to be significantly
assisted by NK cells [24]. The production of activated NK cells (i.e., expressing
CD69 and CD107a) is essential for the success of vaccines. In order to further
evaluate NK cell activity, we evaluated CD3
One month following the booster dose, we identified a unique NK-cell profile
that was enriched for maturing NK “memory” CD56
After one month of a booster dose, CD127 (IL-7 receptor chain) expression on
CD56
NKG2D is an activating receptor essential for the antiviral and anticancer
functions of NK cells. NK-cell CD56
Individuals showed increased Granzime-B synthesis one month after the boost
dose, as well as increased CD69 expression, a hallmark of cell activation [30],
in CD56
The purpose of NK cells that simultaneously express numerous receptors is
unknown; however, it is most likely involved in the activation or inhibition of
NK-cell subsets. It has been noted that SARS-CoV-2 causes an increase in HLA-E
expression [32], which causes NK cells to perform less effectively for protection
against the virus. It’s intriguing to observe that 1 month following the booster
dose, CD57 and NKG2C were substantially expressed on CD56
Activating profiles with enhanced NKG2D
The datasets used and/or analyzed during the current study are available from the corresponding author on reasonable request.
These should be presented as follows: RR, MC, GZ designed the research study. LM collected data and patients. SR, GS, SB, FC, VG performed the research. VG, DB, AP analyzed the data. GZ, AP, RR, MC wrote the manuscript. All authors contributed to editorial changes in the manuscript. All authors read and approved the final manuscript. All authors have participated sufficiently in the work to take public responsibility for appropriate portions of the content and agreed to be accountable for all aspects of the work in ensuring that questions related to its accuracy or integrity.
The study was conducted in accordance with the Declaration of Helsinki, and approved by the Institutional Review Board of the Area Vasta Emilia Centro della Regione Emilia-Romagna (CE-AVEC) with the number 122/2021/Oss/AOUFe. Informed consent was obtained from all subjects involved in the study.
We thank Iva Pivanti for technical support.
This research was funded by University of Ferrara grants (5x1000; Crowdfunding 2021; FIR2021).
The authors declare no conflict of interest.
Publisher’s Note: IMR Press stays neutral with regard to jurisdictional claims in published maps and institutional affiliations.