- Academic Editor
In this article, we reviewed the use of photodynamic therapy (PDT) for breast cancer (BC) in animal models. These in vivo models imitate the cancer disease progression, aid diagnosis, as well as create opportunities to assess treatment during the approval process for the new drug. BC ranks first among women’s cancers. Nowadays, there are many diagnostic methods and therapy options for BC but the majority of them have severe side effects. This article discusses the advantages and some disadvantages of the use of small and large animals used for BC models. A literature review showed that the majority of studies have used large animal models, and recently there has been more interest in developing BC in small animal models. BC cell lines such as MCF-7, BT-474, MDA-MB-231, and 4T1 are commercially available for two-dimensional and three-dimensional in vitro cell cultures and subcutaneous models. The purpose of this article is to discuss the performance of PDT in animal models and its further clinical implications. PDT is known to be a non-invasive therapy, which uses monochromatic light and energy to excite photosensitizers (PSs) for the generation of reactive oxygen species as the required factors. Herein, we discuss the use of five photosensitizers in BC models such as chlorin e6 (Ce6), methylene blue, indocyanine green, 5-aminolevulinic acid, and meta-tetra(hydroxyphenyl)chlorin. The database PubMed and Scopus were searched for keywords: ‘photodynamic therapy’, ‘breast cancer’, ‘animal model’, ‘clinical studies’, and ‘photosensitizer(s)’. The PDT search results in animal experiments and its effect on a living organism indicate the possibility of its application in clinical trials on women with local and disseminated BC. The availability and accessibility of small and large BC animal models enable the progress and trial of cancer drugs for innovative technologies and new diagnostics and treatments.
Cancer is the main problem of 21st-century medicine. Animal research is the basis for the development of new forms of cancer treatment. The appropriate animal model is selected based on the type of cancer, the purpose of the study, the cost, and the time required to develop the model. Moreover, the similarity to human diseases in terms of the appearance of metastases, stages of the disease, and processes in the immune system should be taken into account [1]. Among the large number of animal models used in breast cancer (BC) research, rats and mice are the most common, due to their genetic and physiological homology with humans (about 98% genetic similarity), and therefore, widely used in research on nanoparticles (NPs). The results obtained in these murine models are highly reproducible [2, 3, 4].
BC tumors are determined by the timing of screening, correct diagnosis, and medical intervention [5]. BC is the most common cancer and has the highest mortality rate in the Eastern and Western world. Breast cancer ranks first among diagnosed tumors. In the USA, the incidence of BC increased from 0.102% in 1980 to 0.142% in 1999, and a ~0.131% decrease from 2011 to 2017 [6, 7]. An extensive group of women where BC ranks first is Chinese women, accounting for approximately 2% of newly diagnosed BCs and are the cause of nearly every 10 deaths [8]. In India, the incidence of BC has surpassed cervical cancer, causing the most deaths to date, and is the leading cause of cancer mortality [9]. In Europe, one in three cancers diagnosed in women is BC [8]. In total, BC accounts for more cancer deaths among American women than even lung cancer [6]. For instance, the incidence of BC in the USA was 12.9% in 2017 [6, 7]. Breast cancer is the principal cause of female cancer in Europe and higher socioeconomic status has been linked to higher occurrence [10].
Yedjou et al. [11] analyzed the effect of racial differences in the American population on BC and inferred that younger Black women have more invasive BC, and is characterized by a higher mortality rate than in White women, which might be related to barriers to early health care access, detection or screening, and different way of treatments. Furthermore, different gene pools have a strong influence.
The World Health Organization (WHO) histologically distinguishes 19 subtypes of breast cancer [12], among them ductal carcinoma (70–75% cases) and lobular carcinoma (10–14% cases) are the most common [12].
The BRCA1 gene was cloned in 1994 by Miki and his team [13]. This gene is located on the long arm of chromosome 17 (17q21). It is a large gene comprising 80 kb of DNA [14] and encoding a protein of 1,863 amino acids. BRCA1 is a suppressor gene that controls the cell cycle through mechanisms such as apoptosis and DNA repair. Its role is to maintain the stability of the genome [15]. BRCA1 is a transcription activator, an element of the DNA double-strand break repair system, and participates in chromatin remodeling in the SWI/SNF complex [16, 17]. In large animal models, correlations between genes and phenotypes may be easier to explain. In addition, human medical equipment such as magnetic resonance (MR), computed tomography (CT), endoscopes, brochoscopes, and many surgical instruments can be successfully used.
There is no doubt that the years 2020 and 2021 passed under the pandemic COVID-19. Since the beginning of the pandemic, the number of cancer diagnoses, including BC, has decreased, which does not imply that the incidence has also decreased. Furthermore, diagnostic breast imaging, surgical consultation, genetic research, and treatments including radiation therapy, chemotherapy, and surgery have also reduced during the pandemic. Yin et al. [18] noted a decline in the average weekly imaging of 94.6%–61.7%, surgery of 20.5%, surgical consultation of 11.5%, and genetics consultations dropped to 39.9% in the period from February to April 2020. The reason may be due to the limited access to medical services but moreover, Vanni et al. [19] proved that fear of COVID-19 infection delayed further procedures upon BC appearance. All medical data show an increase in the percentage of newly detected, untreated, and metastatic BC over the next few years [20].
The most common treatment for breast cancer is the surgical removal of the tumor. During the procedure, a margin of non-cancerous tissue (the so-called healthy area) is also removed to minimize the risk of recurrence and metastasis. Although the biopsy procedure is well tolerated by patients, it may cause bleeding or damage to organs in the vicinity of the punctured organ. Another method of treatment is radiotherapy, and one commonly used radiotherapy is brachytherapy. Brachytherapy is a procedure that includes placing radioactive sources near the tumor or in the area where the tumor was surgically removed. Despite its high efficiency, it requires a large dose of radiation. A relatively new and emerging therapeutic method is photodynamic therapy.
Photodynamic Therapy (PDT) appears to be a promising alternative in the localized treatment of breast cancer [21, 22]. PDT is a minimally invasive way of treating gastrointestinal cancers and precancerous lesions. Since it is known that photosensitizers (PSs) accumulate selectively in BC, PDT may be able to selectively destroy neoplastic tissue foci, while maintaining surrounding healthy tissues surrounding [23]. PDT can be used in the case of multifocal lesions and repeated without the risk of cumulative toxic effects. Moreover, the therapy is well tolerated by patients and delivers an excellent cosmetic effect in the case of skin lesions [24]. The antitumor activity of PDT includes its effects on the immune and inflammatory response of the body, the direct cytotoxic mechanism, and the indirect mechanism of the occlusion of blood and lymph vessels [25].
PDT is achieved by a photodynamic reaction that is induced by the excitation of
photosensitizer exposed to light [26, 27, 28, 29]. PDT uses light with low and medium
energy values [29, 30] and commercially available PSs. The minimum amount of light
can be delivered to a tissue depth of 1 cm [31]. PDT action can take place in two
ways. In a type I reaction, the excited PS reacts with biomolecules such as
lipids, proteins, and amino acids to form superoxide radicals by electron
transfer. In a type II reaction, the excited PS gives singlet oxygen
(
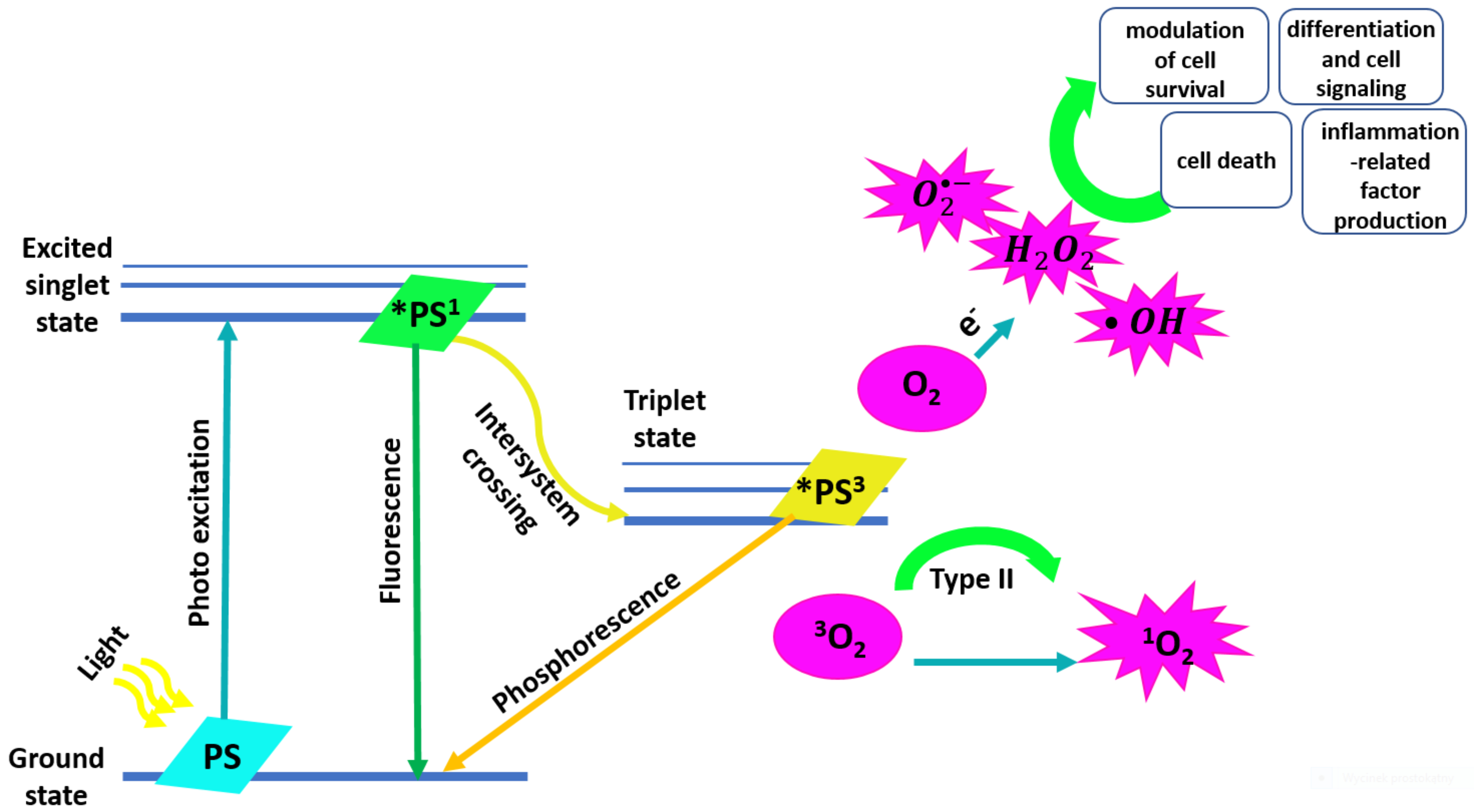
Mechanism of reactive oxygen species (ROS) generation by photodynamic therapy and their influence on the cell death and differentiation, cell signaling and inflammation.
Delivery of PS to the tumor occurs either actively by targeting molecules for
the uptake and delivery in specific tumors, or passively, by enhancing the
permeability of cell membranes [35]. Reactivity with ground-state molecular
oxygen (
Review articles, research articles, and short essays from the PubMed and Scopus databases were analyzed from their first appearance in the literature until 2022. The selection of articles includes animal models and clinical trials on the use of photodynamic therapy (PDT) in breast cancer (BC). The databases were searched for the keywords “photodynamic therapy”, “breast cancer”, “animal model”, “clinical trials” and “photosensitizer(s)”. This systematic review is based on The Cochrane Collaborative Recommendations and Preferred Reporting Items for Systematic Reviews and Meta-Analysis (PRISMA). The articles were selected based on their relevance to the topic. The basic criterion was free-access articles and articles written in English or Polish (Fig. 2). The article aims to review the use of PDT in the treatment of BC on an animal model and the results of clinical trials available in the literature, which is a consistent continuation of the manuscript presented by us at the level of in vitro studies.
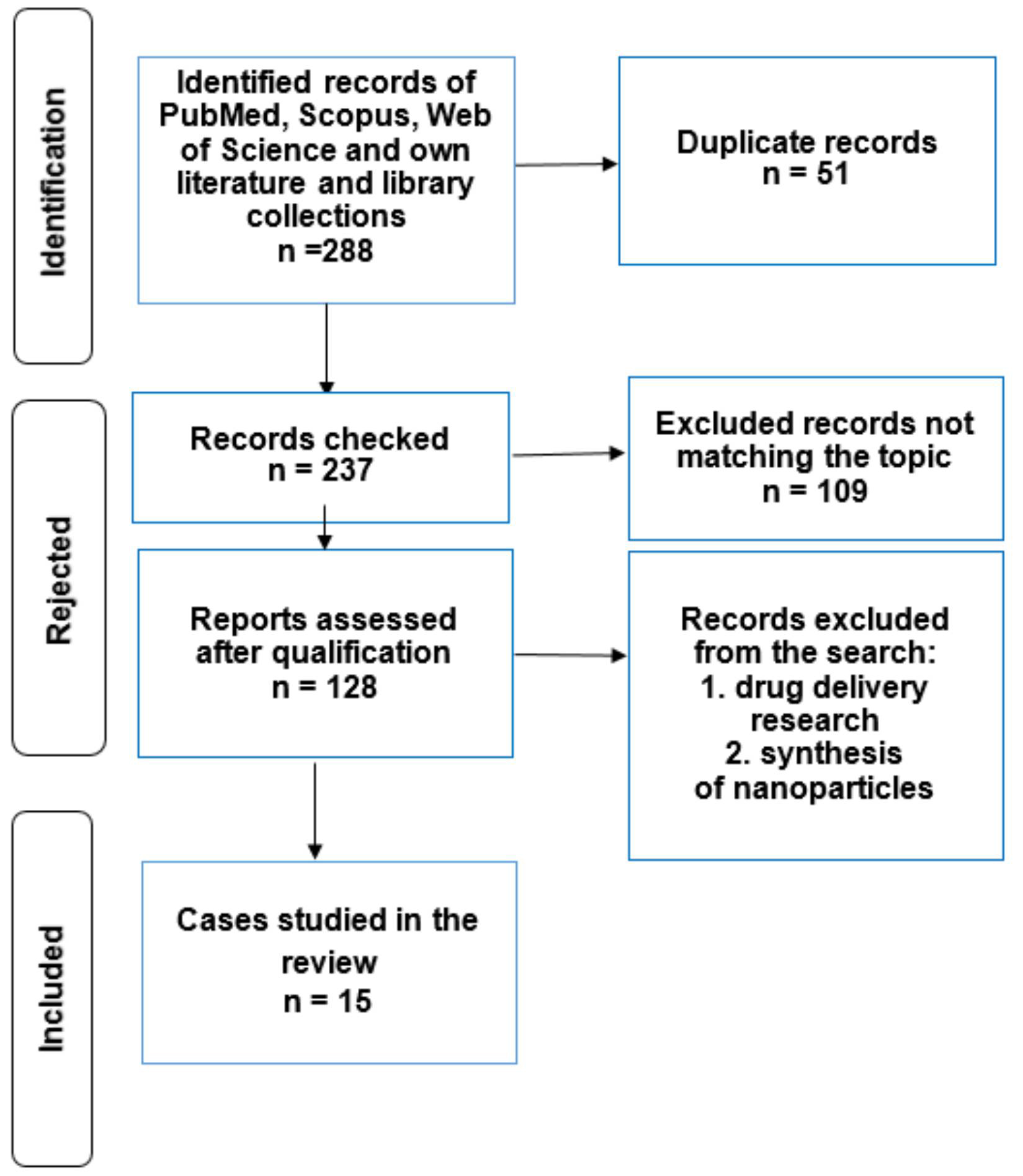
Preferred reporting items for systematic reviews and meta-analysis (PRISMA) statement.
In this review, we consider existing models employed for the pre-clinical use of
nanomedicines for BC treatment—taking in both in vitro two-dimensional
(2D) [36] and three-dimensional (3D) [37], and animal models. The advantage of
nanomedicine is the increase in the concentration of the drug in the tumor
tissue, while limiting the amount of the drug in non-cancerous cells [38]. Table 1 (Ref. [39, 40, 41, 42, 43, 44, 45, 46, 47, 48, 49, 50, 51, 52, 53, 54, 55, 56, 57, 58, 59, 60, 61, 62, 63, 64, 65, 66, 67, 68]) presents PSs excited by light of the appropriate wavelength
that does not damage healthy tissue. Generated
References | Photosensitizer(s) | Wavelength [nm] | Fluence [mW/cm |
[39, 40] | Chlorine e6 | 660 | 100 |
[40] | Chlorine e6 | 635 | 100 |
[41] | Chlorine e6 + NPs | 600 | - |
[42] | Chlorine e6 + NPs | 638 | 300 |
[43] | Photodithazine |
- | 100 |
[44] | Chlorine e6 | 652 | - |
[45] | Methylene Blue + NPs | 660 | - |
808 | - | ||
[46] | Curcumin + NPs | 450 | - |
[47] | 5-aminolevulinic acid | 633 | 105 |
[48] | 5-aminolevulinic acid + NPs | 630 | - |
[49] | Hexaminolevulinate | 450–490 | - |
510–540 | - | ||
[50] | 5-aminolevulinic acid | 633 | 250 |
[51] | Indocyanine green | 808 | 140 |
[52] | Indocyanine green + NPs | NIR | - |
[53] | Phthalocyanine | 690 | - |
[54] | Ryboflavin | 975 | - |
[55] | 5-aminolevulinic acid | 400–700 | 100 |
[56, 57] | Benzoporphyrin | 690 | 150 |
[58] | Benzoporphyrin | 690 | 150 |
[59] | Verteporphyrin | 690 | 100 |
[60] | Chlorine e6 + NPs | 655 | 2 |
[61] | 2-(1-Hexyloxyethyl)-2-devinyl pyropheophorbide- | 658 | 90 |
[62] | Benzoporphyrin | 690 | - |
[63] | Photofrin | 630 | - |
[64] | Pulitin | 660 | 150 |
[65] | Porforin sodium | 630 | - |
[66] | Photofrin | 630 nm | - |
[67] | Mono-L-aspartyl chlorin e6 | 664 | - |
[68] | Mmeta-tetra(hydroxyphenyl)chlorin | 652 | - |
NP, nanoparticle; NIR, Near-Infrared Light.
Technological progress in using light in treatment has contributed to the development of innovative techniques in medical sciences using spectroscopic methods [69, 70]. Chemiluminescence is an analytical method that tracks drugs in the body. It allows the diagnosis and understanding of cellular processes in real-time. Chemiluminescence is the emission of light methods resulting from a chemical reaction. For instance, a luminol derivative coupled with boron-dipyrromethene (BODIPY) can be used to obtain a glow in the form of green light.
Luminescence consists of the emission of electromagnetic radiation with an intensity greater than the intensity of thermal radiation at a given temperature and with a finite duration of lighting that does not disappear immediately after the excitation is interrupted [71]. Luminescence includes photoluminescence—caused by electromagnetic radiation, e.g., by a laser or employing a halogen, xenon, deuterium, or other lamps; electroluminescence—caused by an electric field, e.g., in the flow of electrons in a p-n junction; chemiluminescence—glow occurs as a result of excitation by chemical reactions [72]; bioluminescence—caused by biological processes; radioluminescence—caused by ionizing radiation; X-ray luminescence—glow occurs as a result of X-ray excitation; triboluminescence—occurs as a result of excitation by friction and electrostatic forces; sonoluminescence—caused by ultrasonic radiation; cathodoluminescence—excitation by electron stream; electrochemiluminescence—caused by chemical processes and electric field and thermoluminescence—caused by increasing the temperature.
Breast cancer (BC) animal models are classified by implantation site into three classes: generation of primary tumors [73], generation of experimental metastasis [74], and classification by host immunological state [75]. BC also is classified by the origin of implanted cells in syngeneic mouse models [76], cell-derived xenografts [77], and patient-derived xenografts [78]. Moreover, spontaneous murine breast cancer models are also known as genetically engineered mouse models [79] and carcinogen-induced models [80].
The popularity of PDT testing is related to the design of animal models of disease. Many efforts have been made to study PDT in animal models. The use of animals for PDT purposes is important in medical research. The remarkable anatomical and physiological similarities between humans and animals, especially mammals, have prompted researchers to investigate a wide range of mechanisms and evaluate new PDT treatments in animal models. However, not all results obtained in animals can be directly translated to humans. There exist fundamental differences between the BC model in large and small animals in the parameters affecting the absorption, distribution, metabolism, and excretion of the drug. The main difference between large and small animals is the mass, of approximately 50 kg on average, which affects the accuracy of the results and pharmacokinetic differences.
Breast tumor xenografts are performed in immunodeficient mice.
Most BC cell lines have been recovered from metastatic lesions or isolated from pleural effusion. The reason for this phenomenon is the difficulty of obtaining lines from solid tumors. Less-invasive BC cell lines are underrepresented [81]. Cell-derived xenografts enable the analysis of nanomedicines. Widely used cell lines in pre-clinical studies are MCF-7 (luminal A) and MDA-MB-231 (triple negative breast cancer (TNBC)). To minimize the risk of graft-versus-host disease (GvH), immunocompromised animals are used to implant human BC cells. Unfortunately, genetic mutations, differences in telomere dynamics and regulation, and differences in ligand-receptor interactions result in imperfect animal models [82, 83, 84]. Mouse BC are significantly less hormone-dependent, due to the lower number of cellular estrogen/progesteron receptors (ER/PR) receptors [85]. The types and grades used to classify female BC are not the same as murine BC owing to the difference in the detailed morphological structure of both tumors [86, 87]. The respiration rate is one of the most significant differences between humans and mice, in which smaller animals consume more oxygen per cell. Notably, this factor leads to the induction of hypoxia-sensitive genes and changes the kinetics of its growth in large and hypoxic tumors [88].
Mark Greens proved that monoclonal antibodies inhibit ErbB-2 receptor expression in breast tumors in vivo [89]. New technologies are an opportunity to improve models of BC carcinogenesis [90]. Animal models should be characterized by high reproducibility and represent the most suitable model of the human tumor [91, 92]. Considering the above shortcomings of existing animal models and the fact that their tumor genetics do not perfectly match, it is essential to monitor tumor growth. Therefore an experimental system providing controlled conditions and allowing reproducible experimental set-up, as well as the quantification of processes is required. Molecular differences between tumors are more visible in 3D models than in 2D models, in which prototypes of cell cultures such as cell co-cultures, advanced 3D cell cultures, and patient-derived cells can be analyzed.
The general maintenance of cells to design animal models of BC is described here
[91, 92, 93]. Briefly, BC cells line MCF-7, BT474, or MDA-MB-453 were packed in a
sterile tissue transport medium at the time of surgical resection. The tumors
were dissected under aseptic conditions using scalpels. The samples were formed
into small pieces up to 2 mm
Cells were centrifuged and cultured in medium with antibiotics (density 1–2
Nowadays, research using animals arouses extreme emotions. However, it is necessary to thoroughly understand the safety, stability, and direction of biochemical processes of anti-cancer drugs in animal models, which possess similar prototypes to the human body regarding pharmacodynamics/pharmacokinetics and distribution of the substance. The use of new technology can also advance drug discovery and the development of individualized treatment strategies.
Duanmu et al. [98] analyzed chlorin e6 (Ce6) (Fig. 3) treatment of
chemoresistant BC xenografts in nude mice. Tumors were irradiated with a
635 nm fiber-coupled diode laser (100 mW/cm
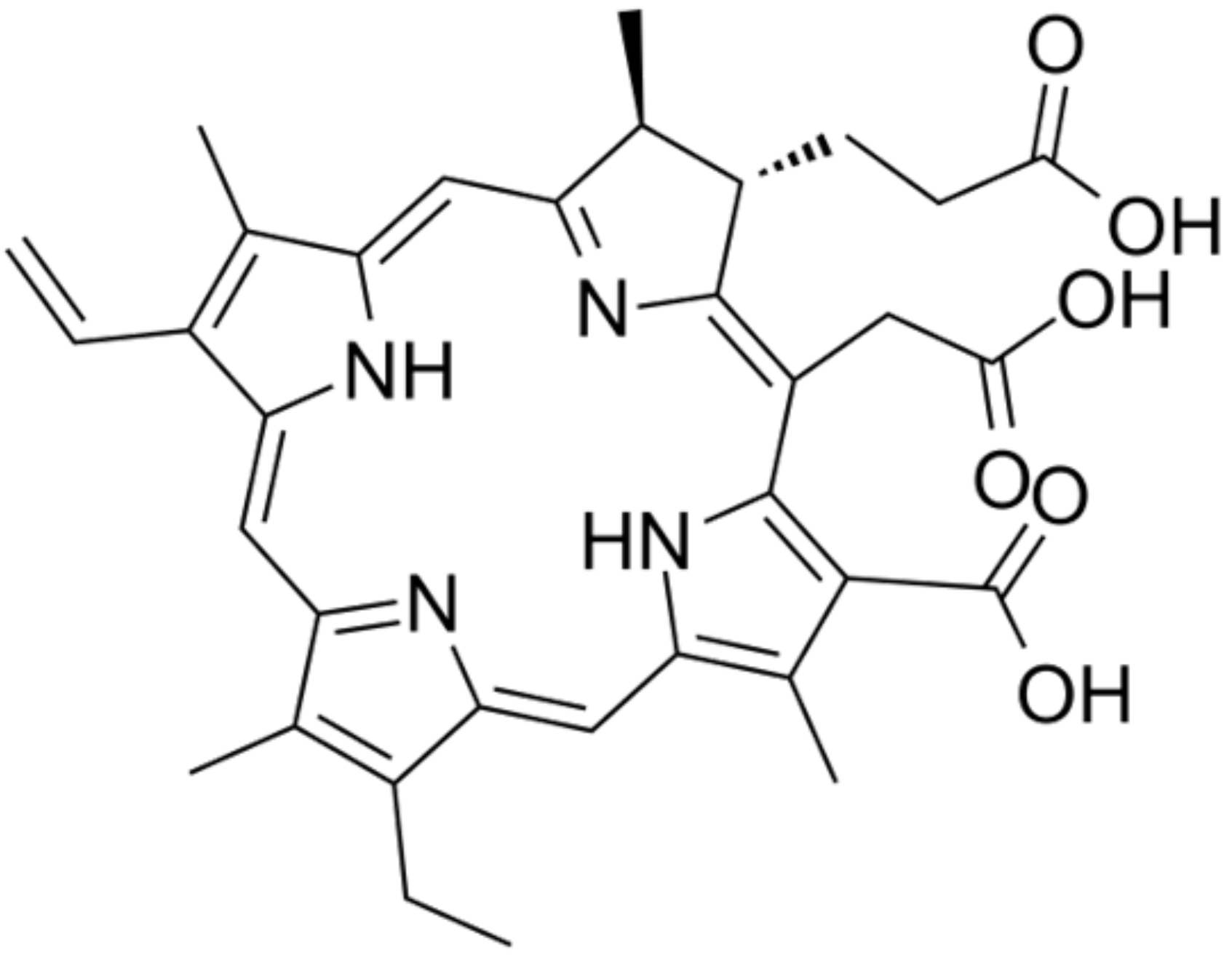
Chlorin e6 (ce6).
Li et al. [99] have used DOX and Ce6 as the model chemotherapeutic drug and PS, respectively, which were encapsulated. The in vitro and in vivo study used a connection of chemotherapy Doxorubicin (DOX), photothermal therapy PTT (NIR), and photodynamic therapy (PDT) (Ce6) with a positive anti-cancer effect and no-side effect [98, 99]. Yu et al. [100] created laminarin-based nanoplatforms (HLDM) to deliver PS (Pp IX) in BC, and performed in vivo and in vitro research. A wavelength laser was used as the light source. DNA destruction, nuclear lysis, and subsequent death of MCF-7 BC cells were observed in vitro. Micelles showed greater phototoxicity under light conditions than in its light. In vivo study showed that Pp IX-loaded HLDM micelles could effectually deliver PS into tumor cells and produce ROS-mediated, subsequently reducing tumor volume and overcoming instability of Pp IX [100].
Mesoporous silica NPs (MSNs) have facilitated the potential for drug delivery
system (DDS)P+ due to several characteristics. NPs that are similar to red blood
cells (RBC) were created for transporting DOX to BC by using Ce6 in PDT. To
evaluate the PDT effect on DOX in vivo, tumors were treated by the 655
nm laser at 2 W/cm
The collagen in the tumor also reduces the effectiveness of the treatment. Tang
et al. [101] observed that after initial treatment with losartan,
in vivo collagen (type I) level was reduced by 53% compared to placebo.
Further, the combination of losartan with the Ce6-PMO nanoplatforms showed
therapeutic efficacy and an increase in the suppression rate of tumor volume to
up to 82% [103]. To enhance photothermal therapy, polydopamine (PDA) was
synthesized with TiO2 nanoparticles. Then, synergistic phototherapy nanoprobes
were constructed by coupling Ce6 with Mn
Accordingly, the indicator M30/SPF may be useful in monitoring tumors after PDT
[37]. Kim et al. [106] produced cancer-targeting peptide p
18-4/Ce6-conjugated polyhedral oligomeric silsesquioxane (PPC) NPs for improving
the targeting ability of Ce6 to BC cells, thereby enhancing PDT
efficacy. In vitro and in vivo studies confirmed their
accumulation in the tumor and inhibition of BC growth [106]. Rollakanti
et al. [51] proved that Vitamin D boosted the performance of PDT in a
small animal model of BC. Mice were injected subcutaneously into MDA-MB-231 BC
cells. Tumors were preconditioned with calcitriol, 1 µg/kg i.p (low and
safe dose) once for 3 days. PS used 5-aminolevulinic acid (ALA) and the tumor
received 250 J/cm
Many studies have shown that nanographene oxide conjugated with different PS are
effective PDT/PTT agents against different tumors. Dos Santos et al.
[46] used methylene blue (MB) to create nanoplatforms to study their effect in
combination with PDT/PTT on murine BC metastasis and primary BC (4T1-Luc). This
experiment used an LED with a wavelength of 660 nm (fluence 90.8 J/cm
Mouse tumor allografts (syngeneic mouse models) are particularly important due to the efficient immune system, undisturbed by the foreign gene pool derived from the tumor. They are particularly relevant for studies of immunotherapies.
Phuong et al. [42] increased the effect of Ce6 by combining it with
albumin nanoparticles (NPs) created from bovine serum albumin (BSA). BC 4T1 cells
from the murine model were irradiated using 660 nm laser light. The complex
displayed a remarkably enhanced tumor suppression effect when irradiated by 660
nm light compared with free Ce6 (tumor volume 90
The expression of tissue factor (TF) was detected in endothelial cells of
pathological capillary blood vessels associated with solid tumors. A study has
proved that fVII is a natural ligand for TF. Liang et al. [43] increased
the antioxidant effect of NPs composed of gambogic acid-grafted hyaluronic acid
(HA-GA) and Ce6. The treatment was carried out in mice with BC composed of 4T1
cells when the tumor was 155 mm
5-ALA has been also proposed as a sonosensitizer for sonodynamic therapy (SDT)
with promising results on BC [108]. Female severe combined immunodeficiency (SCID) mice bearing MDA-MB-231 tumor
xenografts were used for animal model experiments by Fahey and Girotti [48] to
examine the use of iNOS/NO in resistance to PDT. Yu et al. [100] created
nanocycles with Hematin-Laminarin-Dithiodipropionic
Hematin-Laminarin-Dithiodipropionic Acid-MGK, named (HLDM) and Protoporphyrin IX
(Pp IX). In vivo experiments revealed that the Protoporphyrin IX (Pp IX)
-loaded HLDM micelles could induce a remarkable anti-tumor effect, simultaneously
making laminarin a potential drug delivery system (DDS) [100].
Čunderlíková et al. [50] explored the applicability of
hexyl-ester of ALA hexaminolevulinate (HAL) for PDT purging of BM grafts from BC
cells in the murine 4T1 BC model. HAL has greater lipophilic properties, which
makes it easier to pass through cell membranes than ALA. Mice with ex
vivo bone marrow cleansing by HAL-PDT had been shown to possess a lower number
of lung metastases and a longer survival time (the filter combination consists of
a 450–490 nm band-pass excitation filter, a 510 nm
beam splitter, and a 510–540 nm band-pass emission filter) [51].
Gao et al. [103] analyzed whether integrin
Mice with BC 4T1 were used and divided into 4 groups: control, DSAB-HK PDT,
anti-PD-1, and DSAB-HK PDT + anti-PD-1 (70 J/cm
Ahn et al. [110] researched the effects of Cisplatin (Cis) on PDT in BC
using a mouse model. To activate PS (Cis at the concentration of 3 mg/kg), a
diode laser 660 nm/80 J/cm
Ren et al. [112] designed polyethylene glycol (PEG) modified
hematoporphyrin (HPP)-based NPs system to load DOX (HPPD) and achieved a
synergistic effect of chemotherapy and PDT. HPPD/PDT in mice with BC led to
significant tumor ablation with reduced cardiotoxicity. A group of scientists
headed by Subramaniyan [113] considered that a local tumor would be suppressed by
locally released patitaxel (PTX) + PDT and a distant tumor by systemic action.
The rats (Fischer 344) with local large tumors (16 mm) and smaller distant tumors
(4–6 mm) were injected with PTX prodrug (dose: 1 µmole kg
Hypericin (HY) has the advantage of being active in the dark and has a high
tendency to accumulate in primary and metastatic tumors. This feature was proved
by Blank et al. [114] on mice with breast adenocarcinoma (DA3), squamous
cell carcinoma (SQ2) and lung metastases. DA3 survival increased from 15.6% to
34.5%, SQ2 from 17.7% to 46.1% in SQ2 [114]. The concentration of
MDA-MB-231-mCherry cells was set at 2
PDT uses the existence of NPs to clarify and enhance its effectiveness. Brezániová et al. [115] synthesized thermosensitive NPs. These NPs were bound to the PS temoporfin to improve in vivo anti-cancer effect in Nu/Nu mice BC MDA-MB-231 tumors [115]. Cathepsin is a subsection produced by several types of cancer, including BC, which represents their potential as biomarkers for treatment. Ben-Nun et al. [116] created a nanoprobe with PS (qABP) targeting cathepsin. Scientists evaluated the advantages of the probe on cell apoptosis after irradiation (710–760 nm) in mice with 4T1 BC [116]. Liu et al. [117] used liposomal nanoplatforms with platinum nanoparticles (nano-PT) verteporphyrin (VP) to enhance PDT by increasing oxygen delivery. The designed model was analyzed in mouse tumor models in vitro and in vivo. It was concluded that this therapy inhibited primary tumor growth and lung metastasis, with no side effects [117]. Spontaneous regression or spontaneous remission of cancer is one of the determinants of cancer treatment. Understanding the physiology and mechanisms of cancer regression can facilitate the selection and most effective form of treatment. Available literature reveals that selected types of cancer have an increased tendency to spontaneous regression and these cancer types include lung cancer, blood cancer, and one of the most common skin cancers, melanoma [118].
Table 2 (Ref. [98, 101, 109, 112, 113]) summarizes the characteristics of the analyzed animal models along with the percentage effectiveness of the applied PDT therapy.
Type of animal model | Efficiency of PDT | References |
Chlorin e6 photodynamic therapy in mice | 12-fold increase | [98] |
Periodic mesoporous organosilicon nanoplatform loaded with chlorine 6 in mice | administration of losartan and Ce6-PMO increased the effectiveness of PDT compared to treatment with Ce6-PMO alone | [101] |
Nude mice with a breast tumor | increase in efficiency by 27% | [109] |
Rat breast cancer model | increase by 47% | [112] |
BALB/c mice aged 9–10 weeks | treatment with Hypericin cured approximately 35% and 46% of animals that carried adenocarcinoma | [113] |
Dib et al. [119] used the nanotechnology bridged silsesquioxane nanoparticles (BSNs) that have been functionalized with PEG and mannose (PORBSNs-mannose) to target BC in zebrafish embryos bearing human tumors in vivo. After injection of BC cells into the body tumor area of zebrafish, larvae were irradiated by laser light at 800 nm, power 3 W in two-photon PDT. Tumor death was observed indirectly by increasing the caspase 3 concentration [119]. PDT was used with laser ablation to intensify the therapeutic effect in groups of mice with BC. The new method showed superior results to single treatment techniques that use only one of these approaches [120, 121]. Sine et al. [62] administered the anticancer drug 2-(1-hexyloxyethyl)-2-devinyl pyropheophorbide (HPPH) (Ex/Em 410/670 nm) together with calcein and liposomes in mice model and observed its effect. Animals irradiation was performed for 5 minutes with a diode laser at 658 nm. A reduction in tumor volume and a decrease in luciferase concentration were observed [120].
Xu et al. [109] researched PDT in oncotherapies of
gonadotropin-releasing hormone (GnRH) receptors. The combination of zinc
phthalocyanine (ZnPc) with GnRH analogs showed greater specificity for BC cells
in vivo [109]. Morrison et al. [122] utilized continuous
low-irradiance photodynamic therapy (CLIPT) in a Phase I clinical trial with 630
nm laser energy and intravenously administered porfimer sodium as the PS. Cuenca
et al. [67] used a diode laser (light with a wavelength of 630 nm) in
combination with Photofrin (porfimer sodium) as a PS to reduce metastases in the
chest in patients with BC. Tumor necrosis which is photogenerated by
According to the literature, vitamin D deficiency is a sign of photosensitivity. One of the methods to reduce the discomfort associated with photosensitivity is vitamin D supplementation. Physicians most often recommend oral supplementation in doses of 2000 to 5000 IU a day [128].
The main goal of PDT is to destroy malignant (disease/cancer) cells. PDT influence is also observed in the cells surrounding the tumor. There are a few validated studies that highlight the fact that PDT also affects normal cells. However, the undeniable fact is that the evaluation of the normal cells after photoreaction can help in better understanding the principle and mechanism of PDT. There is an example in the literature where human epidermal keratinocytes were used to analyze the effects of PDT treatment. Studies have confirmed that PDT promotes autophagocytosis and induces the apoptotic process of keratinocytes [129].
In the literature, a study on animal models suggests that liposomal aluminum phthalocyanine sensitized many cells to light, a process that was associated with the effect of PDT, i.e., extensive, irreversible apoptosis, the occurrence of which is crucial for the effectiveness of the treatment [130].
Animals have many features similar to humans, particularly in their physiology and behavior. The similarity in the brain structure is reflected in external features. Therefore, there are many scientific studies in which the authors used animal models in analogy to humans. We believe that it is important to develop research on syngeneic models, which, due to their simplicity and lower cost of acquisition, are a promising alternative to the popular xenograft models.
PDT is used in many cancers as a radical, or palliative treatment, offering minimal side effects, and good cancer specificity compared to alternative and conventional oncological treatments, such as surgery, chemotherapy, or radiation therapy. Research is anticipated to enhance PDT as a treatment for BC and to rationally select conventional, and modern combination therapies, such as cancer vaccines, immunotherapy, oncolytic viral therapy, and immunotherapy.
This article highlights both the advantages and some disadvantages of the use of small and large animals for BC models.
The photosensitizers localize in mitochondria, near nuclear areas, and endoplasmic reticulum, making them good candidates for BC PDT. Due to the action of PDT primarily through apoptosis, PDT is characterized by low side effects. However, it is worth considering the conjugation of photosensitizers with other molecules, which is a further challenge in treatment.
Another advantage of PDT is its potential property to induce immunity against recurrence and damage of metastases distant from the treated sites, which provides additional benefits over existing treatments.
PDT can also be used as a complimentary adjuvant therapy but further research is required to investigate if PDT can be used successfully in BC.
On the other hand, the main disadvantage of using PDT in BC is the absence of large randomized controlled trials. Therefore, treatment schedules still need to be improved and standardized to achieve better therapeutic efficacy. The proper selection of the photosensitizer, a suitable wavelength of light, the irradiation duration, and intervals between PS and PDT, would improve the effectiveness and safety of BC PDT. Based on the data collected in our review, we would like to highlight the promising potential of nanomedicine in the treatment of BC. Nanoparticles demonstrated promising in vitro tumor targetability, therefore it is necessary to apply them in studies on animal models to fully understand the pharmacokinetic/pharmacodynamic of PDT.
MCC, AKK, DA and GC designed the research study. MCC, MKO and KD analyzed the data. All authors contributed to editorial changes in the manuscript. All authors read and approved the final manuscript. All authors have participated sufficiently in the work and agreed to be accountable for all aspects of the work.
Not applicable.
Not applicable.
This research received no external funding.
The authors declare no conflict of interest.
Publisher’s Note: IMR Press stays neutral with regard to jurisdictional claims in published maps and institutional affiliations.