- Academic Editors
Tendon is a bundle of tissue comprising of a large number of collagen fibers that connects muscle to bone. However, overuse or trauma may cause degeneration and rupture of the tendon tissues, which imposes an enormous health burden on patients. In addition to autogenous and allogeneic transplantation, which is commonly used in the clinic, the current research on tendon repair is focused on developing an appropriate scaffold via biomaterials and fabrication technology. The development of a scaffold that matches the structure and mechanics of the natural tendon is the key to the success of the repair, so the synergistic optimization of the scaffold fabrication technology and biomaterials has always been a concern of researchers. A series of strategies include the preparation of scaffolds by electrospinning and 3D printing, as well as the application of injectable hydrogels and microspheres, which can be used individually or in combination with cells, growth factors for tendon repair. This review introduces the tendon tissue structure, the repair process, the application of scaffolds, and the current challenges facing biomaterials, and gives an outlook on future research directions. With biomaterials and technology continuing to be developed, we envision that the scaffolds could have an important impact on the application of tendon repair.
Tendon is a bundle of fibrous connective tissues that connects muscle and bone tissues. Tendinous tissues play a pivotal role in musculoskeletal activities as well as help transmit contraction forces from the muscle to the bone [1, 2]. Musculoskeletal disorders are a major health burden worldwide. It is estimated that more than 25% of adults suffer from tendon-related musculoskeletal disorders [3, 4]. Tendon injuries primarily result from high-axis sports, including skiing, basketball, and soccer [5, 6]. Besides, tendon-related injuries mostly occur in the anterior cruciate ligament (ACL), Achilles tendon, rotator cuff, flexor tendon, and patellar tendon, which account for a total of 45% of all musculoskeletal injuries. The probability of re-tear after the repair is about 4.4% for the Achilles tendon, 20% for the rotator cuff, and 30% for the ACL [7, 8].
The success of tendon repair may be related to several factors, such as the patient’s age, activity level, body mass index (BMI), lifestyle, number of tendon injuries, extent of the tear, and so on. For instance, the risk of rotator cuff tears is 2‒3 times higher in elderly people than that in younger adults. Similarly, a high cholesterol level is also associated with an increased risk of rotator cuff tears. Achilles and patellar tendons are thinner and stiffer in smokers than that in non-smokers. The injury site inflammation may drive tendon fibrosis, which may further hamper the healing process [9, 10]. The hypocellularity and hypovascularity of tendons have been shown to be responsible for the spontaneous healing failures following an injury, which lead to the production of disordered extracellular matrix (ECM) as well as weak biomechanical properties of the regenerated tendon tissues [11].
Biofabrication refers to the production of complex biological and non-biological products from live cells, molecules, ECM components, and biological materials [12]. Biomaterials are one of the key factors in tendon tissue engineering (TE). A series of materials, including natural and synthetic polymers as well as therapeutics and cells, in combination with fabrication technology, have been used for tendon tissue repair. Tissue-engineered scaffolds may not only provide structural and mechanical support to the degenerated tendon tissues but may also induce the repair of tendon tissues. Moreover, the degradation rate of the biomaterials may be further tailored to leverage a conducive microenvironment for tendon tissue repair as well as induce the recruitment of endogenous stem/progenitor cells into the injured tissues and facilitate their differentiation into tenocytes for functional tendon repair [13, 14, 15]. Physico-chemical parameters of scaffolds, including stiffness and degradation rate may also influence the behavior of a multitude of cell types, such as the invasion of the scaffolds by stem cells, macrophages, and fibroblasts [16]. Therefore, developing biocompatible and biodegradable scaffolds which can mimic the tendon tissues may be of considerable significance for the repair of injured tendon tissues. In the following sections, we will provide an overview of the tendon structure, its mechanical properties, and the current approaches for tendon repair.
Tendons exhibit a hierarchical structure composed of the bundles of collagen fibers arranged along their longitudinal axis. The fibers of the tendon are organized regularly: the tropo-collagen constitute fibrils, the fibrils constitute fibers, the fibers constitute fascicles, and the tendon matrix is constituted of fascicles (Fig. 1) [17]. In addition, tendon tissues also have horizontal fibers, which can prevent damage and fracture under the action of longitudinal, transverse and rotational forces [18]. The endotendon is a thin membrane composed of lymphatic vessels, blood vessels, and nerves that occupies the spaces between fiber bundles. The epitendon wraps the surface of the tendon and prevents its adhesions to the adjacent tissues [19]. Tendon is mostly composed of the collagen type I (COL I), which accounts for 60% of the dry weight of the tissue and 95% of the total collagen content in the tendon tissues. The other types of collagen components in tendon tissues include collagen type III (COL III), collagen type V (COL V), and collagen type VI (COL VI). At the cellular level, tendon is mainly composed of tenoblasts and tenocytes, which account for about 90‒95% of the cellular components and play an important role in mechanical stimulation. In addition to the tendocytes, tendon tissues also exhibit synovial cells, chondrocytes and vascular cells [20, 21]. While the intrinsic compartment of tendon tissues is mainly comprised of tenocytes and collagen, the extrinsic compartment of tendon tissues connects the synovial-like tissues of the immune, vascular, and nervous systems, which also play an important role in musculoskeletal activity [22].
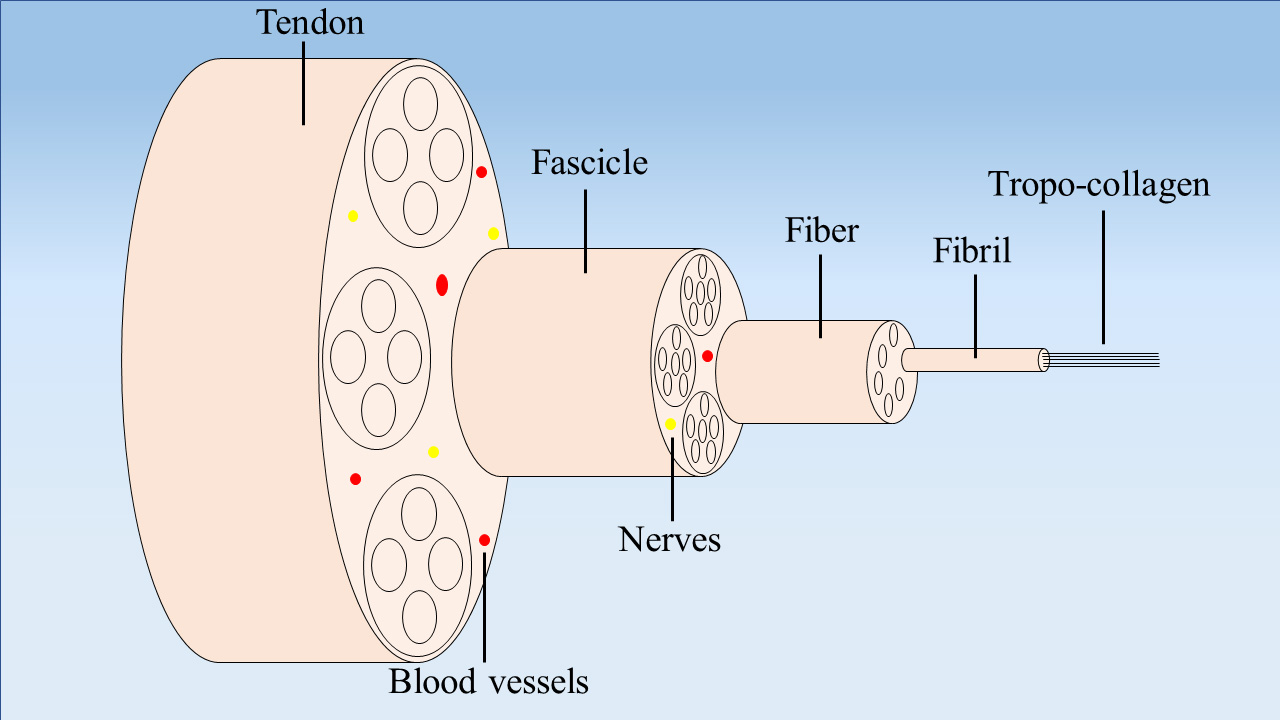
Schematic diagram illustrating the tendon structure. The tendon is composed of tropo-collagen, fibrils, fibers, fascicles, nerves, and blood vessels.
The structure of tendon determines its ability to bear the force. Tendon tissues
are subjected to a large number of tensile stresses in a hierarchical manner. The
macroscopic tensile strength (TS) and elastic modulus (E) of the
supraspinatus tendon are 4.1‒22.1 MPa and 217.7‒592.4 MPa, respectively [23]. The
E of the ACL and Achilles tendon has been shown to be 113
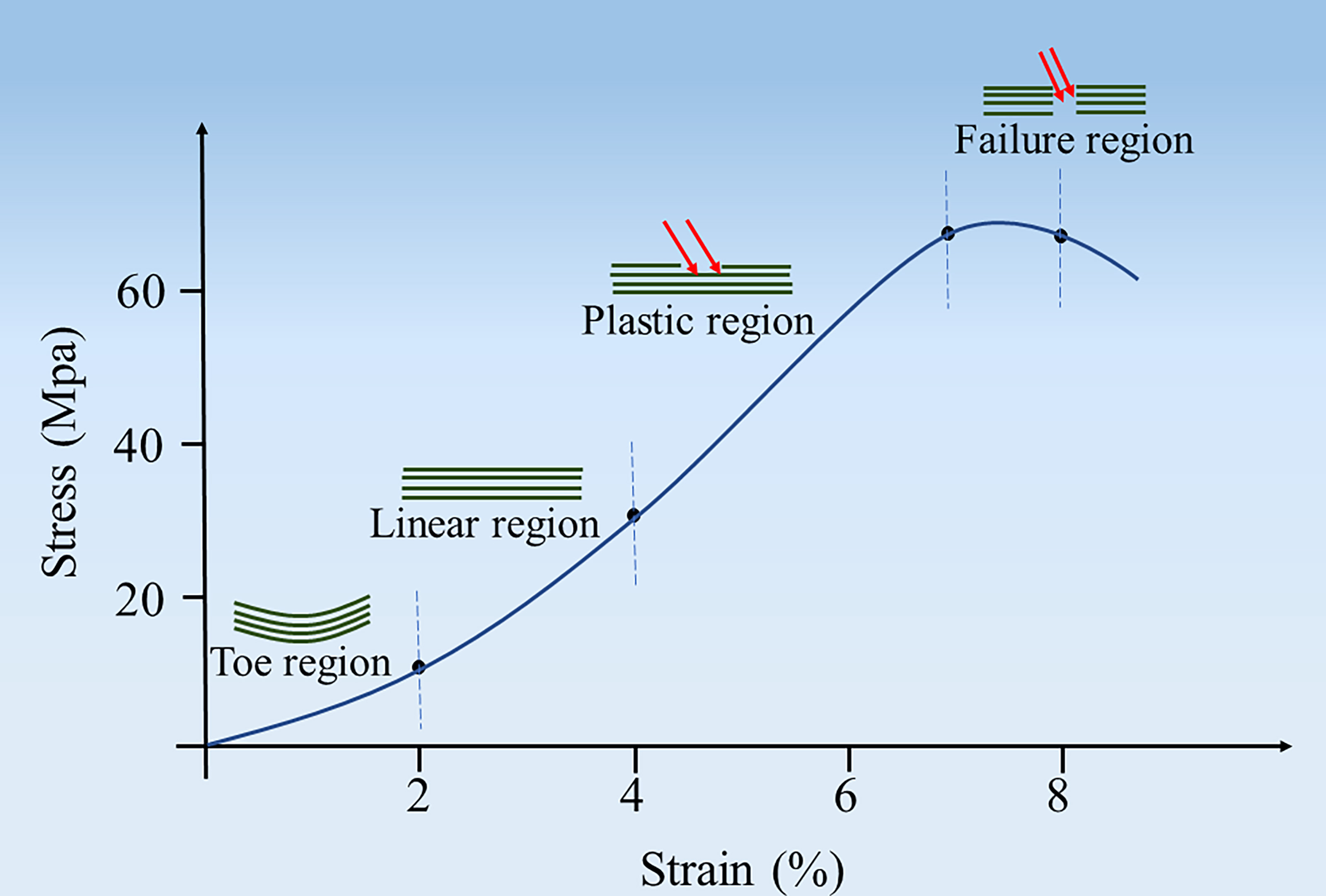
Schematic diagram illustrating a typical stress-strain curve of tendon tissue.
Tendon injuries can develop from chronic tendinopathy to acute tendon rupture. Chronic tendinopathy is usually associated with histological changes, such as increased proteoglycans (PGs) and glycosaminoglycans (GAGs) and disorganized collagen arrangement, but without obvious tissue tearing. However, acute tendon rupture is often associated with direct contusion or tear [26].
There are two modes of repair after tendon injury about intrinsic and extrinsic
healing. The former is the self-healing of injured tendon tissues from the
parenchyma as well as the inner tendon, which may provide better mechanical
properties and reduce complications. Extrinsic healing is mainly caused by
adventitial injury, and there are many problems after injury [27, 28]. Since
extrinsic healing has more problems than intrinsic healing, a lot of attention
has been paid to the process of external healing, and it is divided into three
parts, which are the inflammation phase, proliferative phase, and remodeling
phase [29] (Fig. 3). The inflammation phase occurs 1‒2 weeks following tendon
injury. The blood vessels at the injury site first form blood clots and release
growth factors (GFs) [30], such as transforming growth factor-
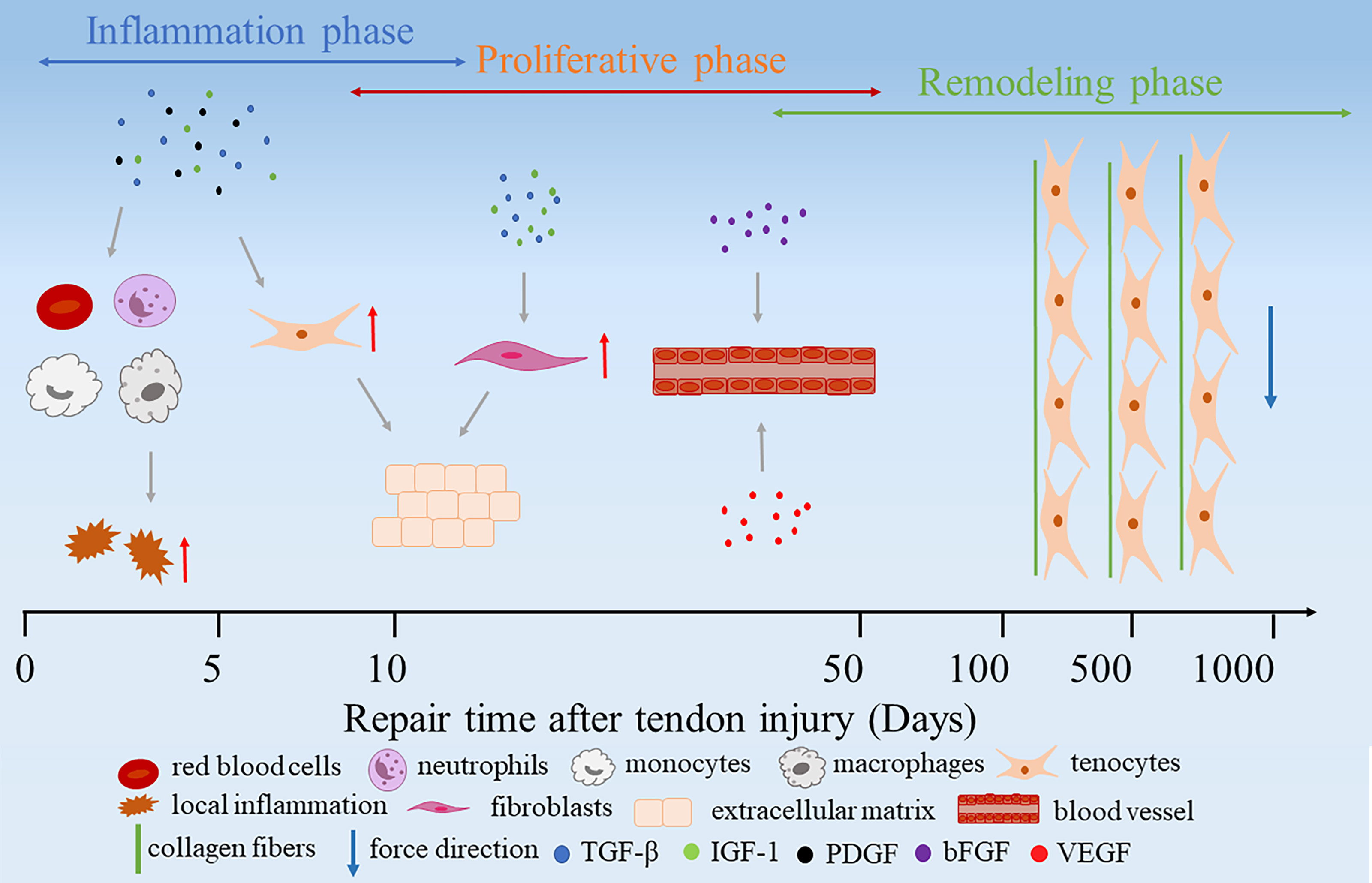
Extrinsic healing of the injured tendon tissues.
After tendon injury, newly generated tissues and the original tendon tissues display varying biomechanical properties due to the different content of COL I and COL III [32]. Consequently, owning to these subtle structural and compositional changes, it is likely to occur several types of problems, which may perturb normal tendon healing. For instance, the degenerative tendinopathy may lead to a lower content of COL I as well as inferior tensile strength and a poor ability to withstand the mechanical load, which may reduce the quality of the tendon tissues as well as compromise their strength. The degenerated tendon tissues display a higher content of COL III, which is smaller and thinner than the COL I, it exhibits insufficient mechanical strength, and may also be prone to the secondary rupture or scarring during the repair process [33].
Peri-tendonious adhesions also pose a major problem after tendon injury within the healing area [34]. The adjacent cells and tissues may stick to the injured tendon. If the repair effect is not ideal, it may lead to loss of tendon structure and failure of tendon repair [35]. However, current treatment modalities do not fully restore the integrity and mechanical functionality of tendon tissues, which are often associated with different types of problems, such as low strength, joint stiffness and poor healing [36]. Tendinopathy may also lead to inflammation of the joint, which causes the occurrence of arthritis [37]. Rotator cuff tears are a common cause of shoulder dysfunction, whose main typical clinical symptom is pain or poor mobility due to inflammation of the synovium [38].
The gold standard treatment option for the injured tendon tissues is reattachment of the tendon to the bone by surgery, but it often leads to the formation of narrowed fibrovascular scar tissues [39]. Autologous tendon transplantation will occur many problems, such as donor-site associated infection, hematoma and local pain [40]. In addition, the poor blood supply to the Achilles tendon may restrict the transportation of nutrients to reach the injured tissue, which may cause several types of problems, such as re-rupture or adhesion of tendon tissues. The induction of neo-capillaries at the injury site as well as improved blood circulation may be beneficial for the recovery of the injured tendon tissues [41]. Various problems will be encountered after tendon injury. Therefore, external interventions are required to promote tendon repair. For example, tendon-replaceable scaffolds have good mechanical properties, local repair materials can prevent tendon adhesion, and scaffolds with optimized properties can promote cell proliferation and differentiation.
While autologous, allogeneic and xenogeneic tendon tissues are often employed to replace the injured tendon tissues, these modalities are limited due to a series of problems, such as the lack of appropriate numbers of donors as well as donor-site associated infection and immunogenicity risks [42, 43]. Recently, a myriad of strategies have been used to promote the repair of injured tendon tissues (Table 1, Ref. [44, 45, 46, 47, 48, 49, 50, 51, 52, 53, 54, 55, 56, 57, 58, 59, 60, 61, 62, 63, 64, 65, 66, 67]). There are many ways to fabricate scaffolds, such as electrospinning and 3D printing, and there are some injectable hydrogels and microspheres, which can be used as GFs and cell carriers, either alone or in combination with other methods for tendon repair [68] (Fig. 4).
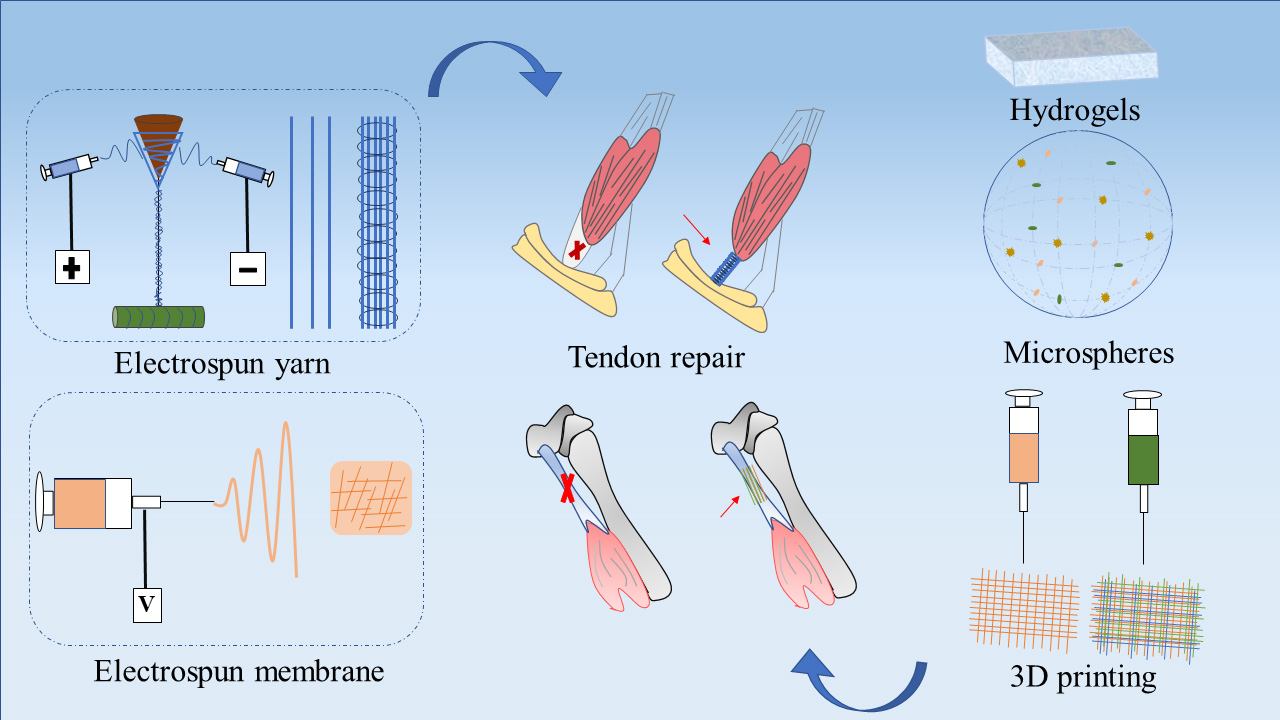
Schematic illustration of the application of different strategies for tendon repair.
Approaches | Materials | Advantages | Application | [Ref] |
Electrospinning | PCL, Hydroxyapatite, ZnO NPs | Cell compatibility, Tendon regeneration, Osteogenesis, Antibacterial | Bone-tendon interface repair | [44] |
HA, PCL, Polyethylene glycol, Silver nitrate, Ibuprofen, Poly (ethylene oxide) | Anti-inflammation, Antibacterial, Prevent fibroblast penetration, Nutrients transport | Peri-tendinous anti-adhesion | [45] | |
SF, PLCL, PCL, PCL microfibers | Cell proliferation, Tenogenic differentiation, Collagen fiber remodeling | Tendon injury repair | [46] | |
PCL, Kartogenin | Chondrogenic and tenogenic differentiation of rat bone marrow stromal cells, Improve the biomechanical properties | Rotator cuff tendon–bone healing | [47] | |
Growth differentiation factor 5, ADSCs sheets, SF, PLCL | Increased cell viability, Tenogenesis-related marker expression | Tendon regeneration | [48] | |
3D printing | Tendon-derived decellularized ECM, Bone-derived decellularized ECM, BM-MSCs, Polyurethane, PCL | Mimics multiple tissue structures and phenotypes, Improve the mechanical strength, Fibrocartilage formation between supraspinatus tendon and bone | Rotator cuff regeneration | [49] |
Polydopamine NPs, TGF- |
Biocompatibility, Nontoxic, Reduce tissue adhesion, Collagen deposition | Tendon repair and prevent tendon adhesion | [50] | |
Ti-6Al-4V powder | Cell proliferation, Thick and ordered muscle fiber, Tendon tissue remodeling, Improve mechanical strength | Tendon growth | [51] | |
PLGA, BM-MSCs, Bone morphogenic protein 12 | Improve collagen organization, Increase fibrocartilage | Rotator cuff tendon–bone healing | [52] | |
PCL, PLGA, Methacrylate gelatin, Methacrylate HA, Autologous ADSCs | Biomimetic recapitulation of native enthesis structures, Improve collagen organization, Support cell communication and infiltration, Show better histological score | Rotator cuff tears | [53] | |
Hydrogel | PCL, Sodium alginate, Melatonin | Promote cell proliferation and viability, Promote the expression of tendon markers, Anti-oxidative | Tendon repair | [54] |
HA, Rhynchophylline | Increase the expression of COL I/COL III, Cell proliferation, Inhibiting the phosphorylation of Smad2 | Tendon healing and prevented adhesion | [55] | |
Chitosan, PLLA | Higher tensile strength, Cell proliferation, Reduce protein adsorption | Tendon regeneration | [56] | |
Alginate, Polyacrylamide, Mesoporous silica | A hierarchically anisotropic structure, Superior mechanical properties | Tendon engineering or replacement | [57] | |
Microspheres | Curcumin, PLGA | Anti-inflammatory, Increase the tensile strength | Achilles tendinopathy | [58] |
Periodontal ligament stem cells, Gingival MSCs, Alginate | Expression for gene markers related to tendon regeneration | Tendon tissue regeneration | [59] | |
Heparin, PDGF | Anti-inflammatory, Gene expression of tendon markers, Increase the collagen content, Improve stiffness and tensile strength | Rotator cuff tendinitis | [60] | |
PRP, Gelatin sponge | Promote BM-MSCs proliferation, Have fibrocartilaginous transition region, High maximum failure load and stiffness | Tendon-to-bone healing | [61] | |
Other strategies | MSCs, PRP | Enhance production of growth factors, Tissue regeneration, Enhance the biomechanical property | Rotator cuff injuries | [62] |
PRP, Graphene oxide | Good biocompatibility, BM-MSCs proliferation, Improve mechanical strength | Supraspinatus tendon reconstruction | [63] | |
VEGF | Regulate the Hippo signaling pathway, Induces angiogenesis | Rotator cuff reconstruction | [64] | |
bFGF, VEGF-A, PLGA, NPs/plasmid complexes | Produce more COL I, New fibrocartilage formation, Improve mechanical strength | Tendon-to-bone healing | [65] | |
Hydroxyapatite/COL I paste | Cell proliferation, Osteogenic gene expression, New bone formation, Sharpey fibers attached to the bone | Tendon-to-bone healing | [66] | |
PET, Calcium phosphate | MC3T3-E1 cells proliferation, Osteogenic differentiation, Improve UTS and stiffness | Anterior cruciate Ligament reconstruction | [67] | |
PCL, Polycaprolactone; NPs, nanoparticles; HA, Hyaluronic acid;
SF, Silk fibroin; PLCL, Poly(L-lactic acid-co- |
The ideal scaffolds for tendon tissue repair should meet the following requirements (Fig. 5): (1) cells can adhesion, grow and migrate on scaffolds [69]; (2) provides nutrients needed for cell adhesion, proliferation and differentiation [70]; (3) no toxicity to cells [71]; (4) materials can degradable [72]; (5) display good mechanical properties to fulfill the requirements of tissue repair: the uniaxial tensile strength should be equivalent to or even higher than that of the strength of the autologous tendon; withstand high-intensity cyclic loads and resist long-term creep deformation, and permit frictionless movement in an efficient sliding to minimize the wear [73].
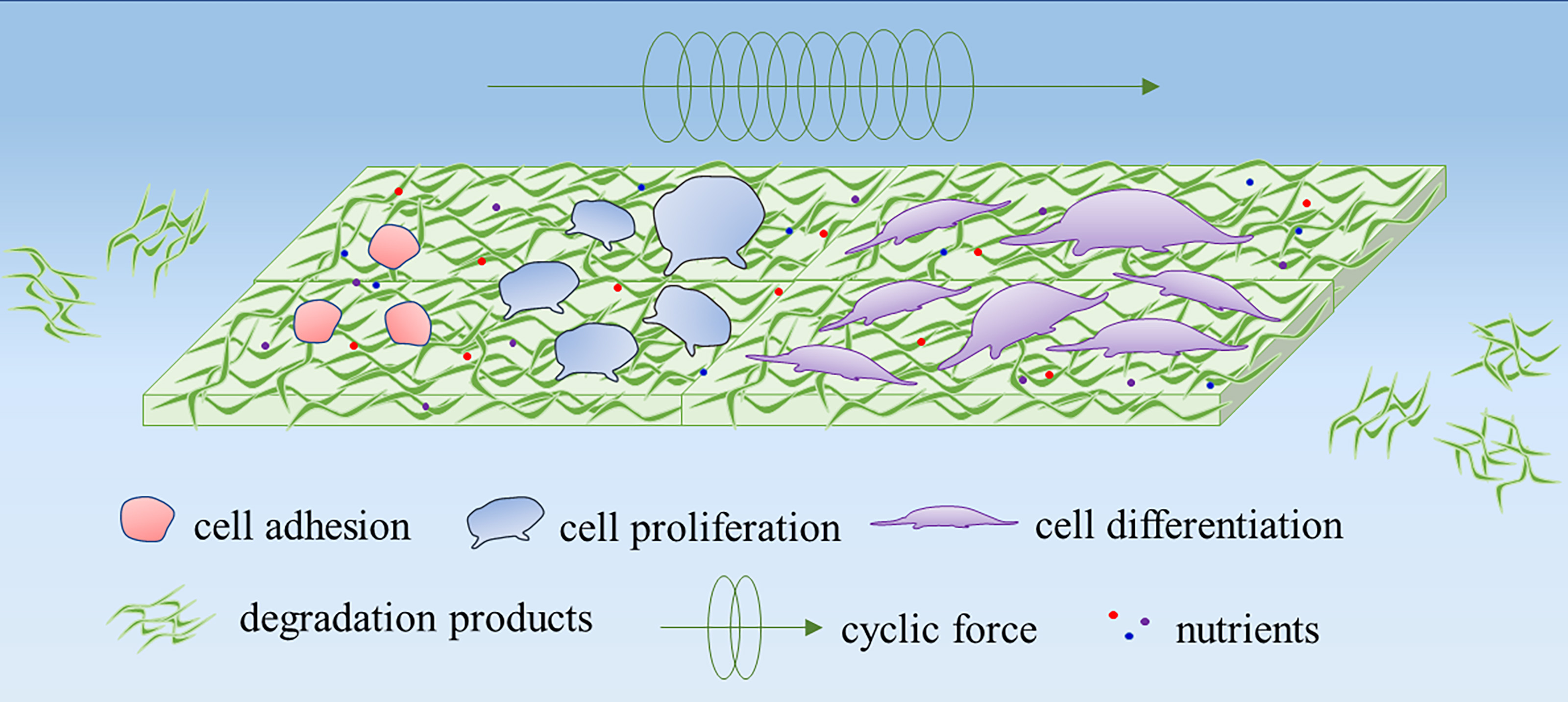
Schematic representation of an ideal scaffold for tendon repair. An ideal scaffold should encourage different cellular behavior, such as cell adhesion, cell migration, and cell proliferation. Besides, the scaffold should exhibit sufficient mechanical properties to withstand the load or reinforce the injured tissues. The degradation products should not induce inflammation at the injury site.
The ideal cell therapy is that cells can refill injured tissues and/or enhance the ability of resident cells. The ultimate goal is to promote tissue reconstruction and functional recovery [74]. It is noteworthy to mention here that several important parameters need to be carefully considered, which may affect cell therapy for tendon repair applications, such as cell type, quantity, and delivery method. Moreover, the choice of the biomaterial may also have a substantial effect on the differentiation of transplanted stem/progenitor cells into tenocytes, including surface morphology, hydrophilicity/hydrophobicity, mechanical strength, and so on [75]. Therefore, the selection of the appropriate cell types with an appropriate biomaterial may lead to better repair of tendon tissues.
At present, cells from various sources are being explored for tendon tissue repair. Among them, mesenchymal stem cells (MSCs) are widely used cell types, which can be obtained from the bone marrow (BM), adipose tissues, and tendon. Additionally, BM-MSCs are multipotent stem cells, which have been shown to differentiate into tenocytes [76]. While mechanisms governing cell-mediated tendon healing yet remain to be explored, it has been reported that the transplanted cells can promote tissue repair through multiple pathways. The transplanted cells may either differentiate into target lineage cells or secrete paracrine factors. The latter can indirectly promote tissue repair, which is manifested by regulating inflammatory reactions and avoiding immunosuppressive treatment after allograft transplantation, and these factors can activate residual receptor cells to play a role in tissue repair [77, 78]. Yu et al. [79] exploited BM-MSCs-derived exosomes for tendon regeneration and increased proliferation and migration of endogenous tendon stem/progenitor cells. Zhang et al. [80] also leveraged tendon stem cell-derived exosomes and observed an enhanced proliferation and migration of tenocytes in a dose-dependent manner. Moreover, the transplantation of exosomes suppressed inflammatory response and promoted the high-quality healing of injured tendons.
Similarly, ADSCs have been shown to promote tendon repair either by differentiating into the tenocytes or by promoting the paracrine effect [81]. From a clinical point of view, ADSCs are easier to be harvested, less invasive and more abundant than that of MSCs [82]. Norelli et al. [83] incorporated ADSCs into hydrogel of collagen and alginate for the subsequent transplantation into the defective Achilles tendon tissues in rats. The cell transplantation led to higher strength and toughness as well as a higher expression of ECM proteins, such as COL I and COL III. It was further revealed that ADSCs injected into tendon defects can survive in vivo even for up to 4‒5 weeks after transplantation.
Tendon-derived stem cells (TDSCs) have also been shown to promote an earlier and better recovery of tendon injury. The transplantation of TDSCs led to increasing collagen content, improved alignment of tenocytes and collagen fibers, formation of collagen birefringence, and improved biomechanical properties of regenerated tissues compared to the fibrin glue-only controls [84]. Xu et al. [85] fabricated PLCL/collagen scaffolds using an electrostatic spinning dynamic water flow system, and then implanted TDSCs on the scaffolds. The mechanical stimulation of the cell-seeded construct was shown to improve tendon tissue formation. The expression levels of COL I, COL III and tenascin C in the cell-seeded constructs were significantly higher on day 14 after mechanical stimulation. In addition, the higher deposition of collagen at weeks 4 and 8 after the implantation of scaffolds significantly improved the maturity and mechanical properties of the regenerated tendon tissues. Similarly, Chen et al. [86] fabricated chitosan scaffolds that by self-deposition and then seeded TDSCs on the scaffolds. The cell-seeded groups exhibited a higher expression of tendon-specific genes as well as COL I production, while a lower deposition of COL III. The organization of COL I, cell density, maximum load and E of the TDSCs-seeded groups were all closer to those of the native group. Consequently, the healing of the Achilles tendon was improved while the adhesion around the Achilles tendon tissues was reduced (Fig. 6, Ref. [86]). TDSCs, as native cells of the tendon tissue, are better adapted to the tendon microenvironment and preferentially differentiate into tenocytes, however, the number of cells in tendon tissue is very small, and the process is more complicated and costly than other cell extractions to obtain a large number of high-quality cells.
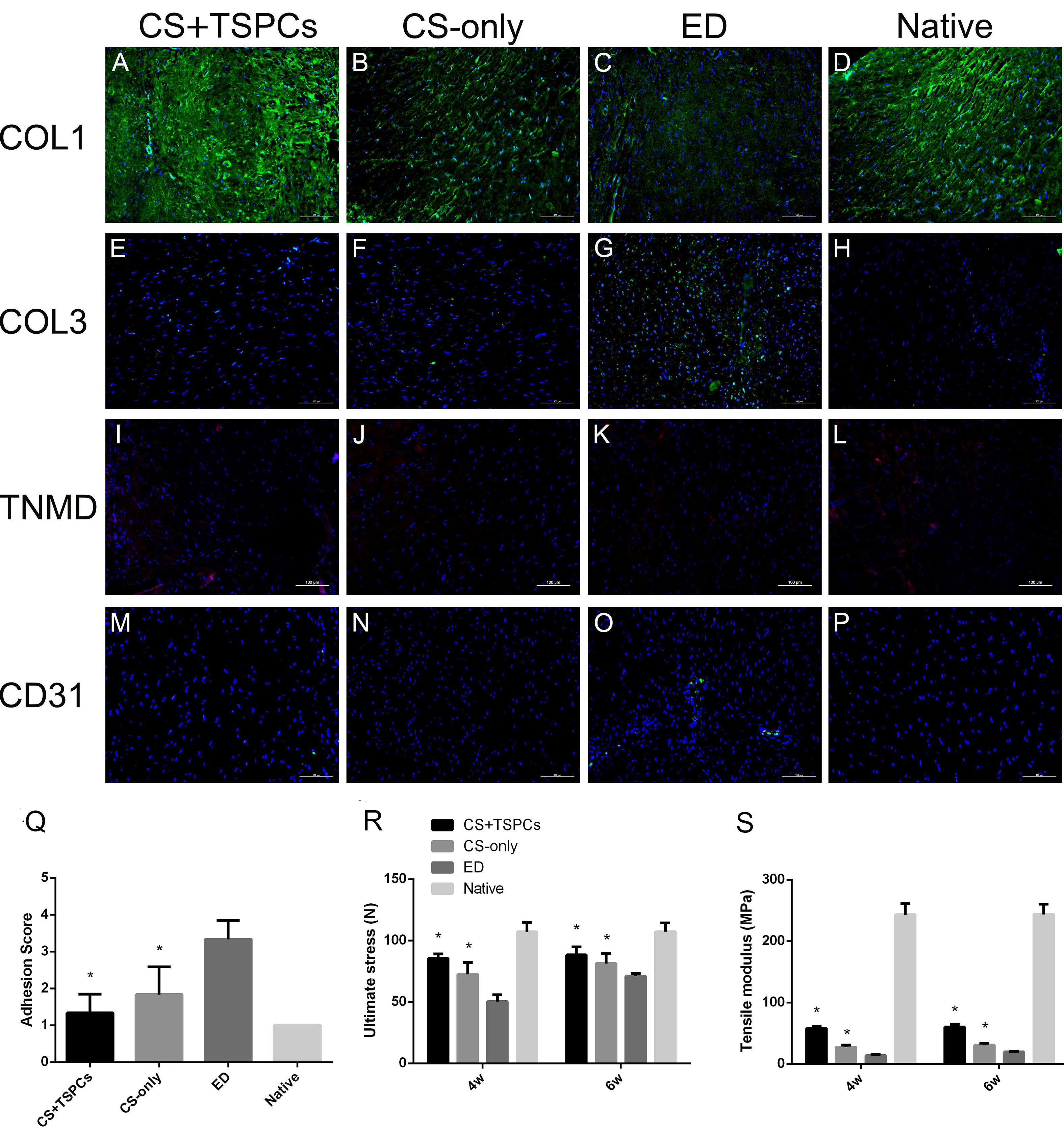
Analysis of tendon tissue by immunofluorescence staining 4 weeks
after the implantation of cell-seeded constructs. (A–D) The production of COL1.
(E–H) The production of COL3. (I–L) The production of tenomodulin (TNMD).
(M–P) the production of CD31. Blue: DAPI (cell nuclei); Green: COL1/COL3/CD31;
Red: TNMD. Scale bar = 100
Electrospinning refers to the jet spinning of a polymer solution or melts in a strong electric field. Under an electric field, the droplet at the tip of the needle changes from a ball to a cone, known as a Taylor cone, and expands into a fibrous filament from the tip of the cone. Electrospinning is a simple, fast, sustainable, size-controllable, and an easy approach, which has already been exploited for the fabrication of different types of scaffolds [87, 88]. Nanofibers resemble the fibrous structure of natural ECM, which may positively influence different cellular behavior, such as cell adhesion, cell migration, and cell proliferation [89, 90]. Electrospinning technology can combine most spinnable natural materials such as chitosan, collagen, SF, gelatin and synthetic polymers such as PLGA, PCL, PLCL, PET, polylactic acid (PLA), polyglycolic acid (PGA) and can load cells and GFs such as BM-MSCs, TDSCs, VEGF to make scaffolds for tendon repair. Electrospun nanofibers can be designed into different shapes and structures, such as nanofiber membranes and nano-yarns, which can also be used in combination with 3D printing technology and hydrogels to meet the needs of tendon repair such as tendon regeneration, tendon-bone healing, and anti-adhesion.
Tendon tissues are composed of coiled nanofibers, however, it is still challenging to fabricate scaffolds that may accurately mimic the microstructure and mechanical properties of human tendon tissues as well as promote the infiltration of endogenous stem/progenitor cells into the implanted grafts [91]. With the advancement in technology, electrospinning can afford the design of scaffolds mimicking the structure of collagen fibrils of tendon tissues, such as three-dimensional scaffolds containing nano-yarns or nanofiber bundles to simulate the multi-layered structure of tendons [92]. Guner et al. [93] fabricated a biphasic scaffold with a randomly oriented core and an oriented shell structure produced by rotary jet spinning and electrospinning. While the shell layer was conducive to cell elongation and orientation, the core layer structure provided a supportive environment for cell infiltration. These scaffolds significantly improved cell adhesion and viability. Sensini et al. [94] exploited high-level nylon 6,6 electrospinning assemblies with single-bundle and hierarchical combinations of nanofibers, which resembled the morphology and orientation of tendon tissues. Moreover, these scaffolds displayed strength and stiffness values comparable to tendons as well as significantly promoted tendon regeneration. Yu et al. [95] fabricated gradient nanofiber scaffolds for photothermal mineralization using an electrospinning setup, which underwent structural change and graded mineralization via the photothermal effect of the indocyanine green. The mineral content increased gradiently in the same direction. The TDSCs were differentiated into tenocytes and osteogenic cells in this scaffold. These gradient nanofiber scaffolds may have potential implications for tendon-bone healing applications. The Angle of simulating tendon structure to promote the repair of injured tendon tissue is one of the hot spots of current research. Although many studies have developed such scaffolds, how to make the whole scaffold oriented is still a difficult problem. For example, the combination of electrostatic spinning and yarns is common. Generally, yarns are oriented, and oriented fiber films can also be obtained by electrostatic spinning. However, how to tightly wrap oriented yarns with oriented fiber films without affecting each other’s structure is a difficult problem. Secondly, if spinning on the surface of an oriented yarn, it is difficult to determine whether the spun fiber is oriented, which is an unstable factor. Future technologies need to do a lot of research to overcome this problem.
The combination of braiding technology and electrospinning technology makes the mechanical properties of the scaffolds superior to those of fibrous membranes, and the adjustable braiding parameters can further help to design customized scaffolds to meet the needs of different tendon tissues, the porous structure of the braided scaffolds is conducive to the infiltration of cells and the transport of nutrients [96]. Barber et al. [97] leveraged PLLA braided nanofibrous scaffolds for tendon regeneration. The mechanical properties of the scaffold mimicked the three-phase mechanical behavior of native tendon under loading. Human mesenchymal stem cells (hMSCs) can adhesion, proliferate, and elongate in a dynamic culture in an in vitro bioreactor. Zhao et al. [98] fabricated MSCs and bFGF-loaded PLGA braided scaffolds. Once implanted into the injured tendon tissues, these scaffolds induced the high expression of tendon-related gene markers. Besides, the structural and biomechanical properties of the regenerated tendon were improved. Similarly, Xie and coworkers [99] developed a braided scaffold exhibiting dynamic mechanical properties as well as spatial adaptability which improved collagen infiltration and remodeling. The regenerated collagen fibers showed curved-like morphology. Moreover, the maturity and structure of the deposited collagen gradually improved with the implantation time. At 16 weeks, the content of COL I was 91.35%, which was similar to that of the native ligament tissues (Fig. 7, Ref. [99]). But it is worth noting that, whether it is hand braiding or machine braiding, it is necessary to consider whether the spun fibers will fall off because of braiding, resulting in uneven distribution of fibers. Secondly, it is necessary to consider whether the braided scaffolds can be cut according to different needs, and whether they will be loose and deformed after cutting. If the scaffold is easy to lose in the process of cutting, then the utilization value is not high.
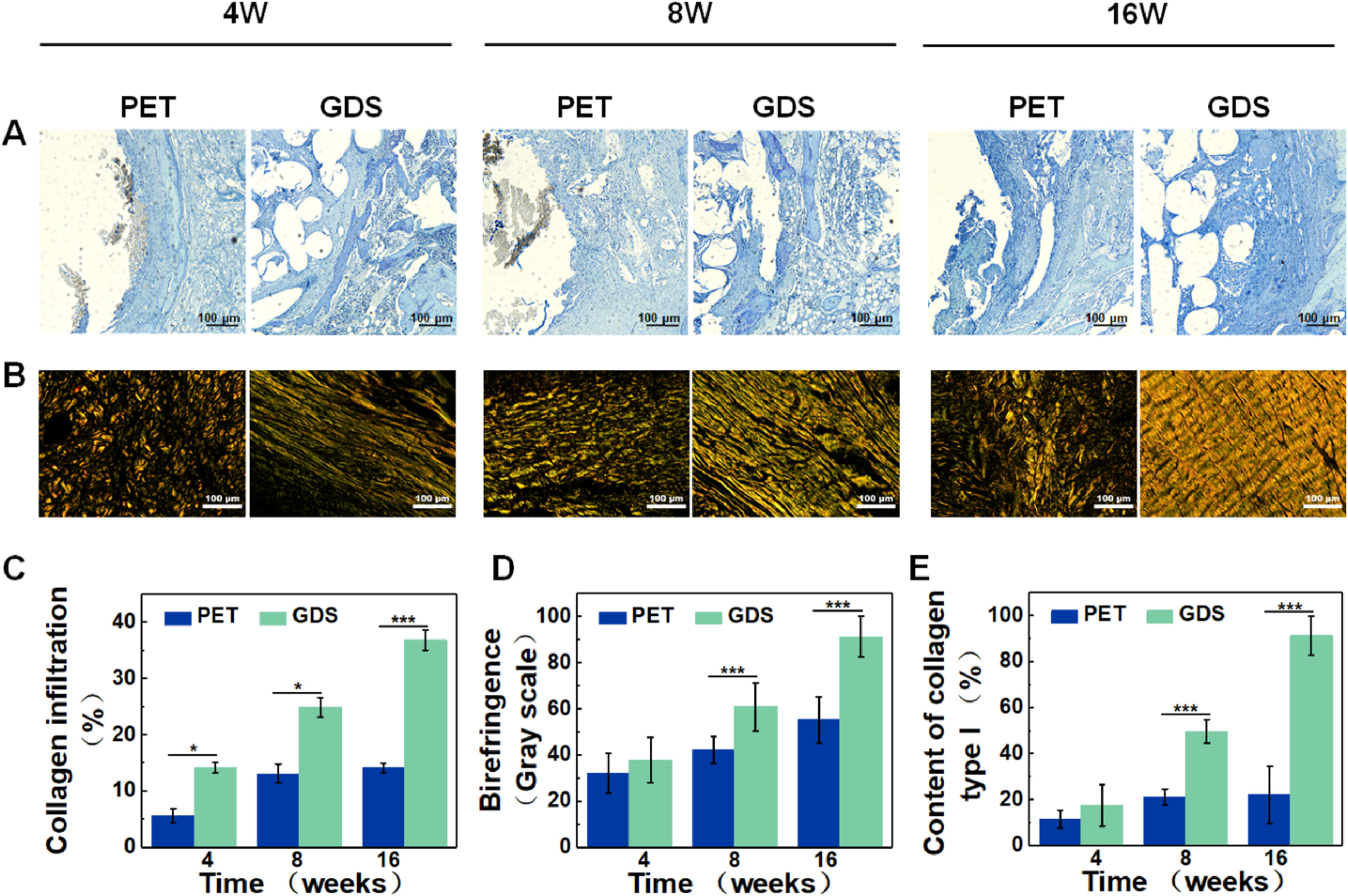
Collagen regeneration evaluation. (A) Masson staining images,
scale = 100
Since adhesion is a common problem after surgery, many researchers have
exploited electrospun scaffolds to reduce tissue adhesion by inhibiting
inflammation for tendon repair. Mao and coworkers [100] prepared a
sandwich-type electrospun scaffold containing ibuprofen and reported suppressed
proliferation of fibroblasts, reduced adhesion of macrophages and lower gene
expression of tumor necrosis factor-alpha (TNF-
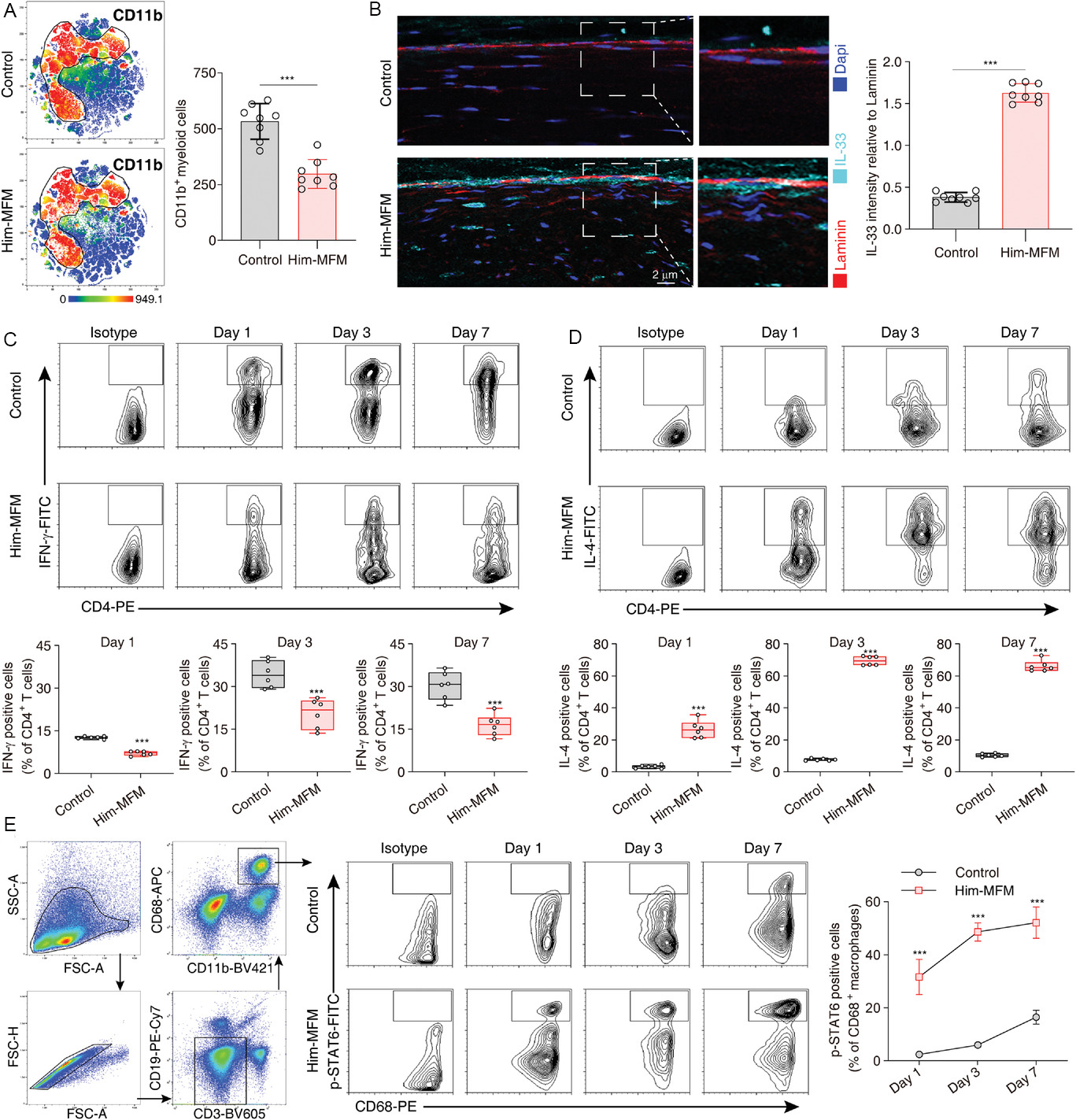
Him-MFM implantation activated mucous-IL-33-Th2 axis for
regeneration. (A) CD11b+ immune cells in control and Him-MFM implantation mice
at 3 days post-injury. (B) Representative fluorescence images of a cross-section
of mouse tendon sheath at 3 day-post-injury in control or Him-MFM group. Scale
bars: 2
3D printing, a type of rapid prototyping technology, is also known as additive manufacturing and has emerged as an alternative method for the fabrication of tissue-engineered scaffolds [102]. 3D printing can create different types of scaffolds with precise geometrical features and pore size [103]. It can better simulate the 3D structure of the ECM and achieve high mechanical properties, and the added GFs and living cells can improve the performance of the scaffold [104, 105]. Consequently, a myriad of approaches have been leveraged to fabricate 3D printed scaffolds and exploit them for tendon repair.
3D printing can be combined with cells to print cell-containing scaffolds for tendon repair [106, 107]. Chen et al. [108] fabricated 3D printed PLGA scaffolds and then seeded BM-MSCs onto them, which exhibited good biocompatibility, promoted collagen formation and increased collagen diameter at the tendon-bone interface, and improved the mechanical properties of regenerated tendons as well as promoted tendon-bone healing. Chae and coworkers [109] used tissue-specific tendon or ligament-derived decellularized extracellular matrix (dECM) components and human BM-MSCs made into bioinks to fabricate 3D printed scaffolds. The pre-treatment of the scaffolds induced the formation of pre-oriented cells and structures 28 days after culture. This in vitro matured 3D tissue structure exhibited better regenerative capacity, promoted the formation of the new tendon as well as led to higher mechanical properties after the ectopic implantation in nude mice (Fig. 9, Ref. [109]). Although 3D printing research has become mature, it is very challenging to meet the requirements of high cell density, high cell vitality and high resolution at the same time. Secondly, a lot of 3D printing on the market is now limited to the realization of macro printing, and the fine control of microstructure is still a difficult point. For cell-carrying printing, it is very important to establish a connection between cells by adjusting the microstructure.
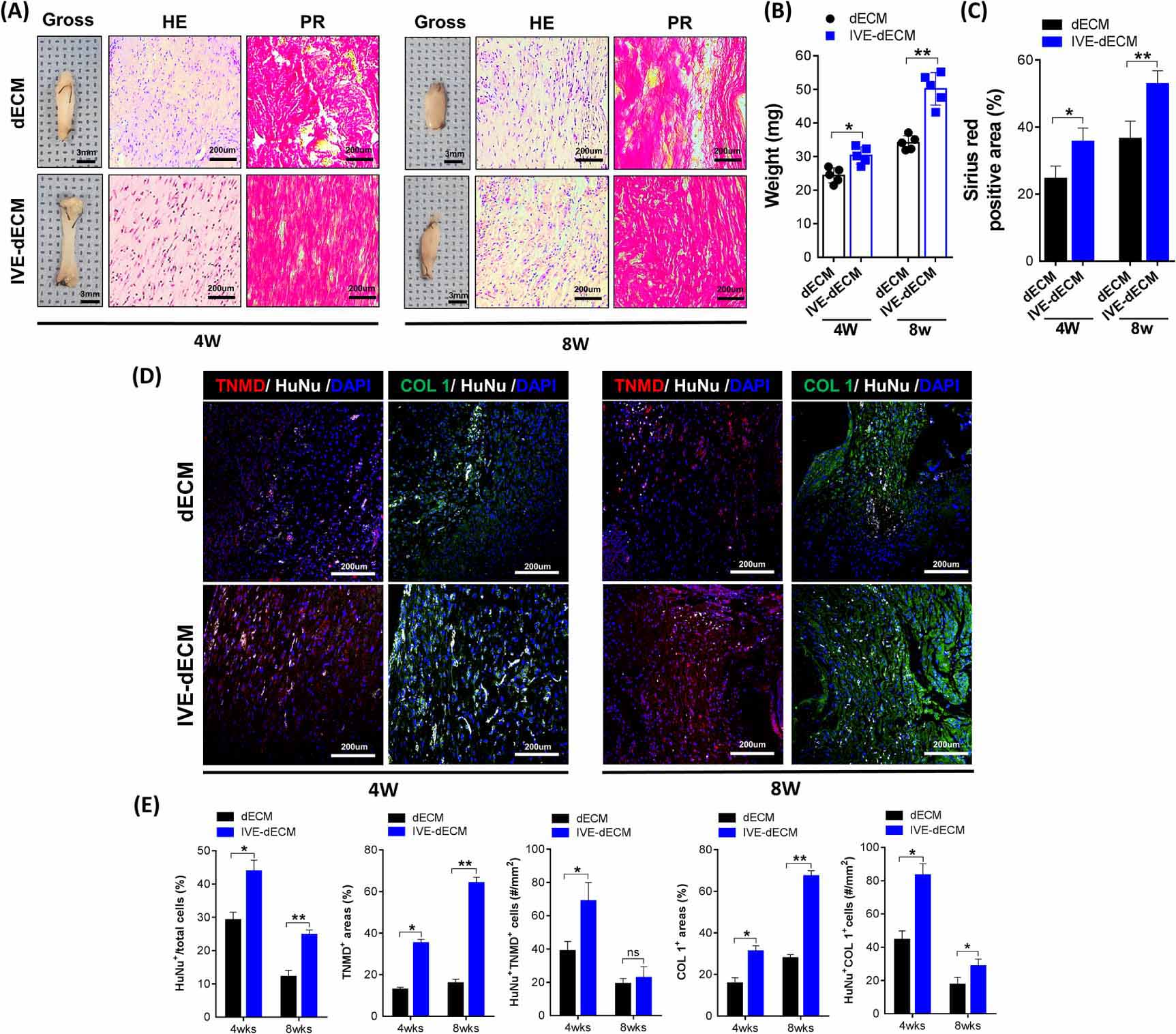
In vivo T/L formation following subcutaneous
implantation of scaffolds in nude mice. (A) Histological analysis using
hematoxylin and eosin (H&E) and Picrosirius red (PR) staining of regenerated
tendon tissues at 4 and 8 weeks postoperatively. (B) Weight of regenerated tendon
tissues. (C) Quantification of PR positive area. (D,E) Immunofluorescence
staining and quantitative analysis results of neo-tendon formation in regenerated
tissues at 4 and 8 weeks postoperatively (scale bar = 200
Tendons are reported to be comprised of about 70% of water content. The weight-bearing collagen fibers are surrounded by a hydrated soft tissue matrix containing PG. In addition, the soft tissue matrix allows collagen fibers to slide relative to each other further avoiding wear-related damage [110]. While hydrogels may contain a large amount of water and resemble biological tissues, they lack sufficient mechanical strength and toughness. However, tendon tissues exhibit high strength as well as excellent toughness. Consequently, to apply hydrogels for tendon tissue repair, it is necessary to remarkably tailor their production methods to improve their biomechanical properties [111, 112]. Nonetheless, hydrogels, especially injectable hydrogels, can be used as drug delivery carriers due to their porous structure as well as high water content and biocompatibility, which can encapsulate bioactive molecules in situ and may be exploited to fill irregular defects [113]. Besides, hydrogels also possess other characteristics, such as easy production and handling, high controllability and operability [114, 115]. Therefore, the improved drug-loaded and cell-loaded hydrogels are widely used in tendon tissue repair applications.
Kim et al. [116] developed an injectable hydrogel containing celecoxib-loaded NPs with an obvious anti-inflammatory effect, which was shown to relieve excessive inflammation during the early stage of tissue injury, promote collagen regeneration, as well as help restore injured tendon tissues. Huang and coworkers [117] developed injectable thermosensitive hydrogels containing kartogenin and mesoporous bioactive glass NPs. These hydrogels were shown to fill tiny fissures after tendon-bone repair, which may also have good application prospects in chronic rotator cuff tears healing (Fig. 10, Ref. [117]). Xu and coworkers [118] developed an injectable fibromodulin-releasing granular hydrogel, which promoted the migration of tenocytes as well as increased the expression level of LOX in tenocytes and accelerated collagen deposition. The implantation of these hydrogels led to functional recovery in the Achilles tendon injury model in rats.
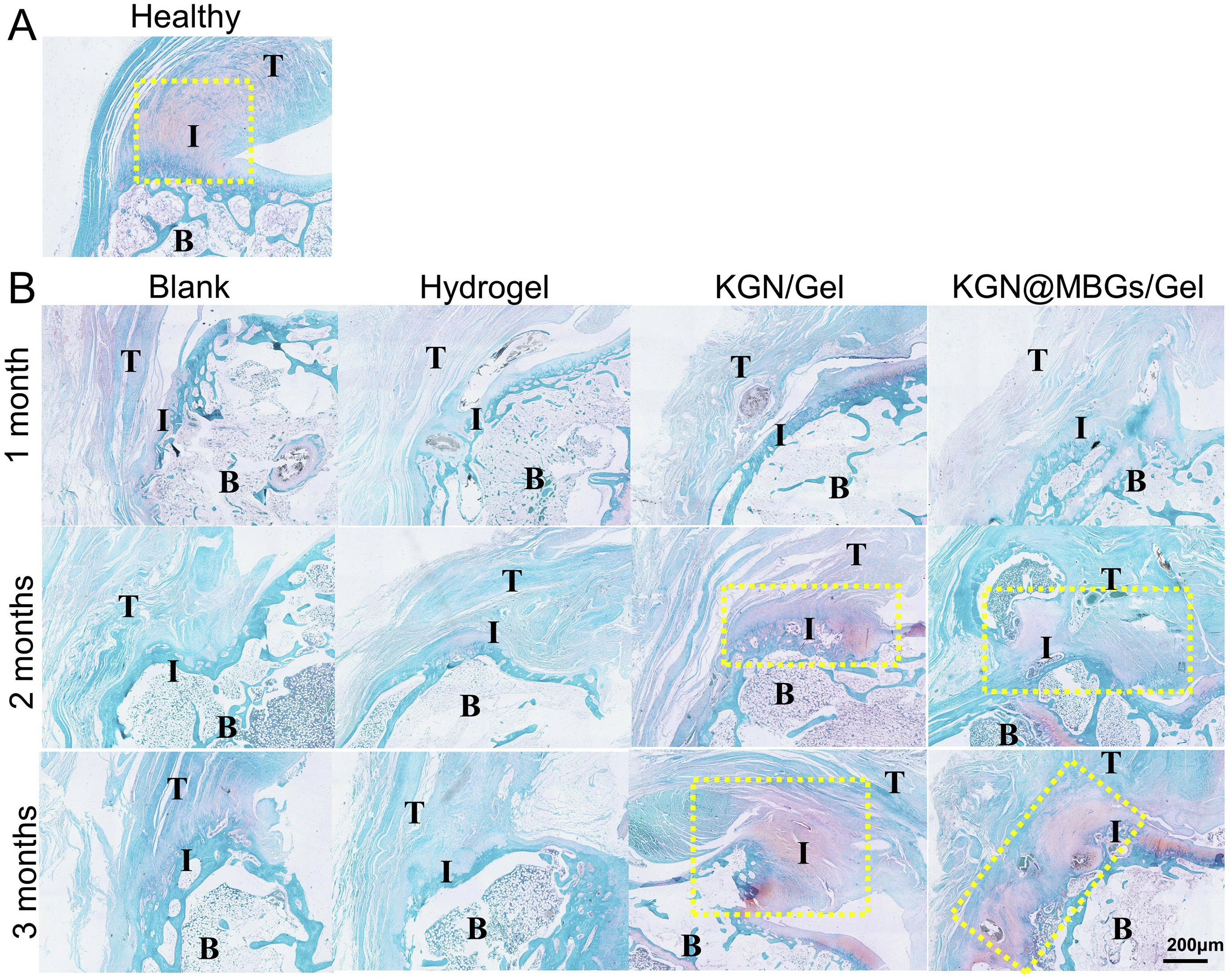
Safranin O-fast green staining results of the interface of tendon-to-bone. (A) Healthy interface presented abundant cartilage layer (red) between tendon and bone. (B) Regeneration of cartilage layer after repair without hydrogel, or with simple hydrogel, KGN/Gel and KGN@MBGs/Gel for 1, 2 and 3 months (T = tendon; B = bone; I = interface; the yellow box labeled the fibrocartilage area). KGN, kartogenin; MBG, mesoporous bioactive glass NPs. Reproduced with permission from [117].
Microspheres are another type of injectable system that has a good clinical
prospect, especially those with inter-connected pores, which may also be good
delivery vehicles for drugs, proteins and cells and may also enable the sustained
and long-term delivery of bioactive molecules, thus providing a minimally
invasive and convenient method for delivering bioactive factors to tendon
injuries [119]. The diameter of the microspheres may be further tailored in the
micrometer range, which may improve drug loading rate and encapsulation
efficiency, and may also prolong the retention of drugs or other cytokines at the
implantation site. Consequently, microspheres are often exploited for the repair
of small-scale injuries, including tendon-bone healing and tendon inflammation
[120]. Microspheres can be assembled from natural polymers, such as chitosan,
alginate, and gelatin, or synthetic polymers, such as PLGA
[121, 122]. Chen et al. [123] designed curcumin-loaded chitosan
microspheres, which promoted the differentiation of tendon stem/progenitor cells
into tenocytes, reduced the progression of tendon ectopic calcification, and
enhanced tendon regeneration (Fig. 11, Ref. [123]). Choi and coworkers [124]
exploited injectable dexamethasone-loaded PLGA microspheres for Achilles
tendinitis healing, which could significantly inhibit the expression of COX-2,
IL-1
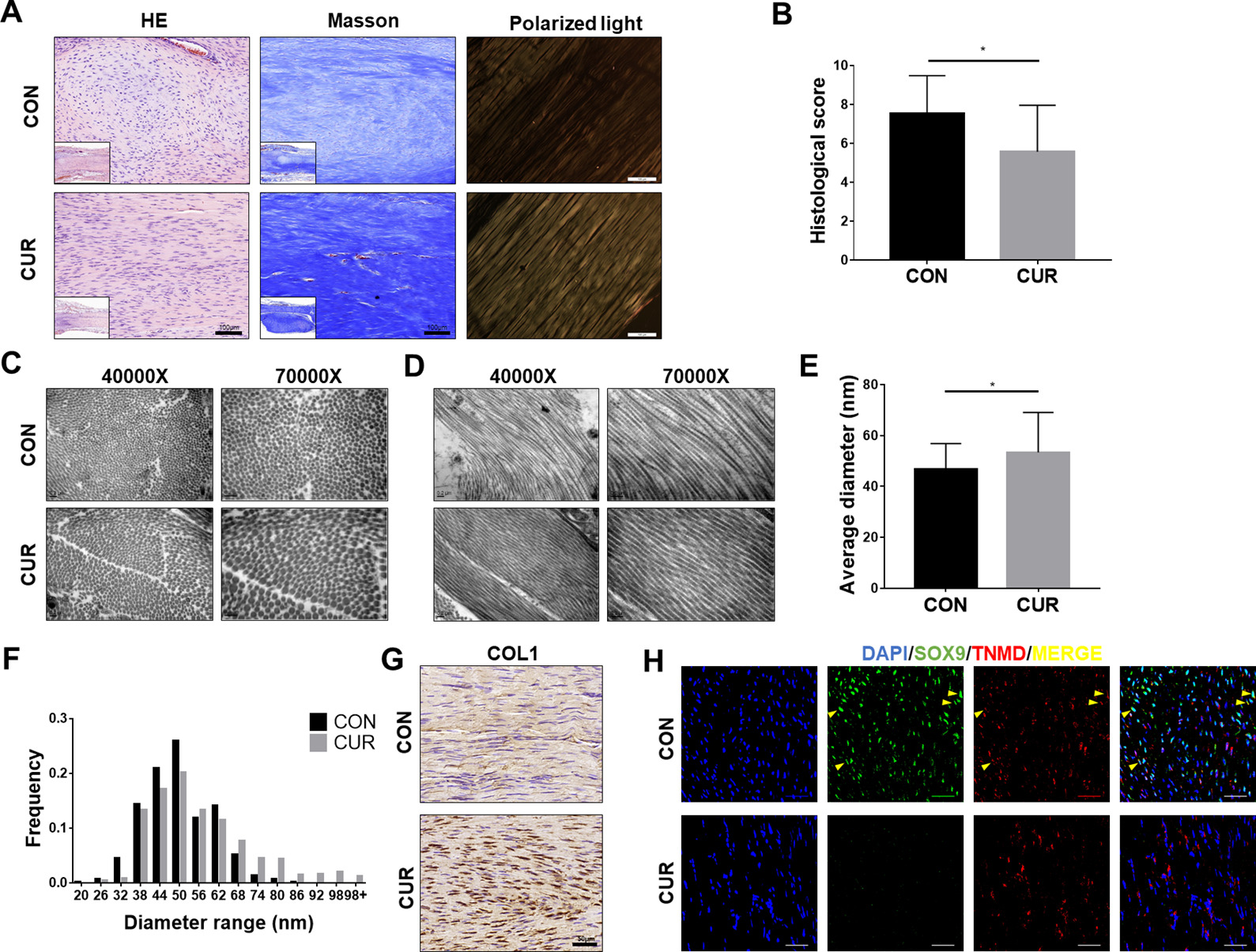
Curcumin contributes to tendon regeneration in rat tendon
calcification after treatment for up to four weeks. (A) H&E, Masson’s trichrome
staining, and polarized light examination of the repaired tissue in the control
and curcumin groups. (B) Histological score of the repaired tendon (n = 5),
*p
Injectable systems are currently used to fill small defects or in combination with other methods. Due to their limited mechanical properties, it is almost impossible to replace the entire tendon as a scaffold. Another issue worth noting is their limited drug encapsulation rate and the problem of drug sudden release needs to be solved.
Besides the strategies described above, a series of bioactive cues has been
leveraged for tendon healing. Platelet-rich plasma (PRP) is the plasma fraction
of autologous blood that contains high concentrations of platelets and GFs, and
upon activation, the PRP may release different types of GFs at the injury site,
including PDGF, TGF-
Since PRP treatment is mainly due to the contribution of GFs, the latter may
also be directly combined with the biomaterials for tendon repair. Some of the
typical examples of the GFs-loaded scaffolds include FGF-2 combined with hydrogel
for tendon repair in chronic tears in rats [131], connective tissue-derived
growth factor (CTGF)-loaded sutures to induce cell infiltration, promote
angiogenesis and collagen deposition [132]. The co-culture of HGF promoted the
proliferation and migration of TDSCs and inhibited osteogenic differentiation
[133]. The PDGF-B improved the viability of BM-MSCs, increased the ultimate
tensile strength and stiffness of tendon tissue, and promoted the expression of
tendon-bone healing-related genes and proteins [134]. The TGF-
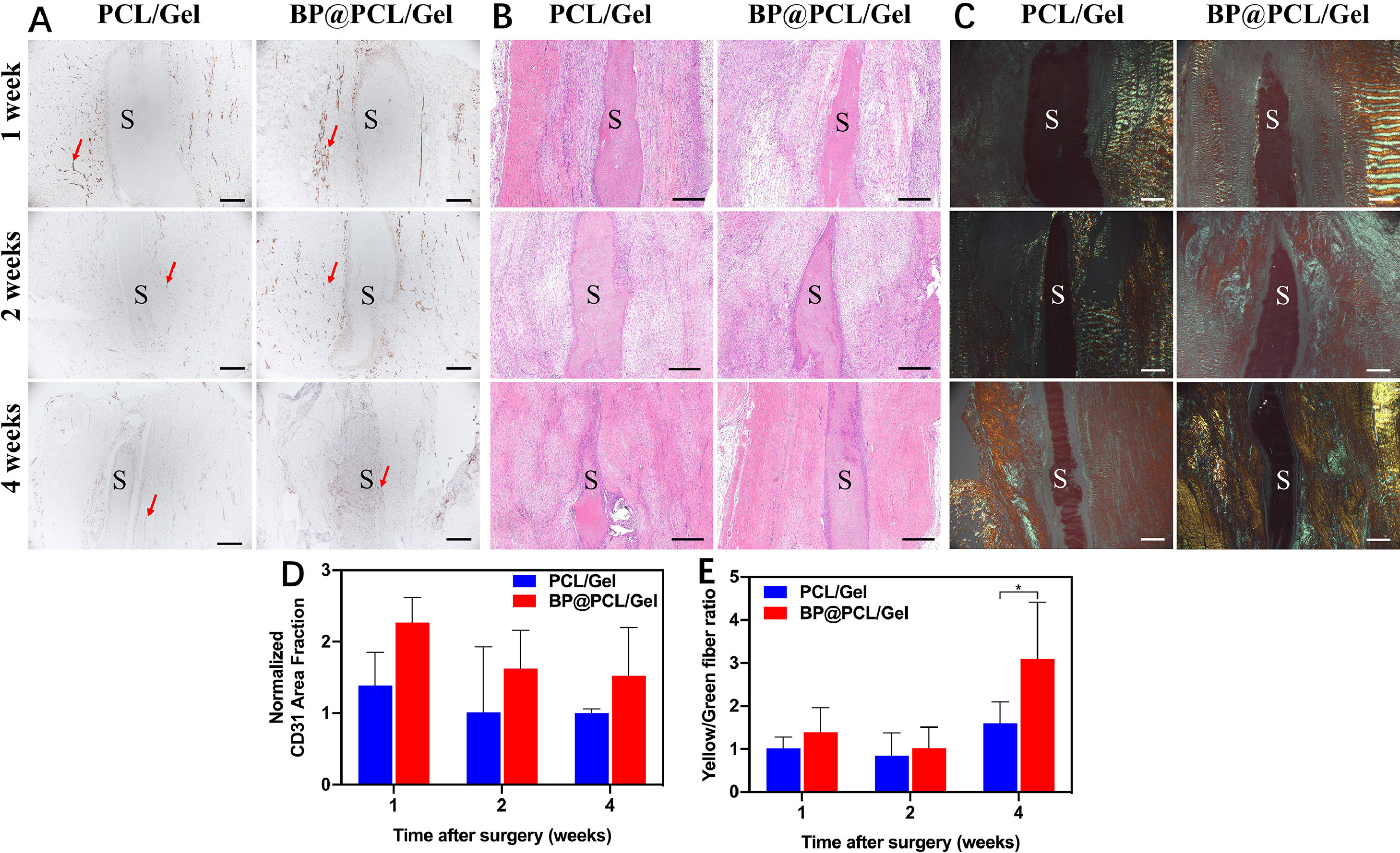
Histopathological analysis of the regenerated patellar ligament
1, 2, and 4 weeks after the implantation of scaffolds. Representative images of
CD31 staining (A), H&E staining (B), and PSR staining (C). Quantitative analysis
of CD31-positive stained area (D). Quantitative analysis of the PSR staining for
the ratio of yellow fibers to green fibers ( n = 3) (E). S = Scaffold. The red
arrows point toward new blood vessels. *p
This review introduces tendon structure, biomechanical properties, repair process, and the application of scaffolds prepared by various techniques for tendon repair. Tendon is a bundle of tissue that transmit the mechanical forces from muscle to the bone without sacrificing its ability to withstand significant amounts of tension. The tendon can repair itself after a minor injury, but when it suffers a major injury, surgical transplantation has to be performed. However, no matter whether it is autologous, allogeneic transplantation, or artificial tendon transplantation, it cannot well meet the clinical needs currently. Tendon repair is a complex process, so the fabrication of scaffolds needs to fully consider all aspects, and it is crucial to mimic the tendon structure and match the mechanics of the original tendon. At present, a series of strategies such as electrospinning scaffolds, 3D printed scaffolds, hydrogels, and microspheres can be used alone or in combination with cells and GFs for tendon repair.
By summarizing the current research, we found that there are still some problems in the application of scaffolds in tendon repair. For example, most scaffolds are limited to characterization in animal experiments and cannot be used in the clinic. As we all know, the characteristics of tendons require scaffolds to have ultra-high mechanical properties, the scaffolds can be stressed without deformation, or the deformation is small and can rebound after deformation, and the loss in this process is extremely small. However, most of the scaffolds with high mechanical properties are non-degradable materials, and the mechanical properties of degradable materials are poor. Therefore, it is an urgent problem to develop degradable materials that can meet the demands of tendon mechanics. The content of cells and blood vessels in tendon tissue is low, and scar tissue will be generated during the repair process. The process of tendon healing is accelerated along with increased angiogenesis. Therefore, promoting angiogenesis is also an important issue in tendon repair. The repair of the tendon takes a long time. After implanting the scaffold, it will be one of the key development directions that the mutual status between the scaffold and the tendon, and the regeneration of the tendon can be observed in real-time. This needs to involve many cross-cutting fields such as chemistry, materials, biology and medicine, and it is difficult to achieve this.
In the future, we believe that researchers need to pay more attention to two aspects. On the one hand, the materials and technology of scaffolds need to be comprehensively considered, to provide mechanical properties, biocompatibility, and implant safety. On the other hand, In vivo tendon repair involves multiple reactions, multiple mechanisms, multiple factors, and the treatment mechanism of scaffolds needs further research to ensure its effectiveness for tendon injury. It is believed that there will be ideal scaffolds for clinical application in the near future.
Study design–XY, XM, MS, BS; Literature search and data collection–XY, JC, YS, WG, ME-N, YM; Data analysis–XY, PC, YC, ZY, ML, HE-H; Writing and original draft–XY, JC, YS; Writing and review & editing–BS, MS, XM; Funding acquisition–XM, MS, ME-N, HE-H, YM. All authors contributed to editorial changes in the manuscript. All authors read and approved the final manuscript.
Not applicable.
Not applicable.
This work was supported by National Natural Science Foundation of China (project # 81501606, 32050410286, and 81771951), Science and Technology Commission of Shanghai Municipality (No. 20S31900900, 20DZ2254900), Sino German Science Foundation Research Exchange Center (M-0263), and Grant-in-Aid for JSPS Fellows (Grant # JP21F21353). This project was also supported by Researchers Supporting Project Number (RSP2023R65), King Saud University, Riyadh, Saudi Arabia. MS is an international Research Fellow of the Japan Society for the Promotion of Science (Postdoctoral Fellowships for Research in Japan (Standard)).
The authors declare no conflict of interest.
Publisher’s Note: IMR Press stays neutral with regard to jurisdictional claims in published maps and institutional affiliations.