- Academic Editors
In the present era of global warming and dramatically increased environmental pollution posing a threat to animal life, the understanding and manipulation of organisms’ resources of stress tolerance is apparently a question of survival. Heat stress and other forms of stressful factors induce a highly organized response of organisms at the cellular level where heat shock proteins (Hsps) and in particular Hsp70 family of chaperones are among the major players in the protection from the environmental challenge. The present review article summarizes the peculiarities of the Hsp70 family of proteins protective functions being a result of many millions of years of adaptive evolution. It discusses the molecular structure and specific details of hsp70 gene regulation in various organisms, living in diverse climatic zones, with a special emphasis on the protective role of Hsp70 in adverse conditions of the environment. The review discusses the molecular mechanisms underlying Hsp70-specific properties that emerged in the course of adaptation to harsh environmental conditions. This review also includes the data on the anti-inflammatory role of Hsp70 and the involvement of endogenous and recombinant Hsp70 (recHsp70) in proteostatic machinery in various pathologies including neurodegenerative ones such as Alzheimer’s and Parkinson’s diseases in rodent model organisms and humans in vivo and in vitro. Specifically, the role of Hsp70 as an indicator of disease type and severity and the use of recHsp70 in several pathologies are discussed. The review discusses different roles exhibited by Hsp70 in various diseases including the dual and sometimes antagonistic role of this chaperone in various forms of cancer and viral infection including the SARS-Cov-2 case. Since Hsp70 apparently plays an important role in many diseases and pathologies and has significant therapeutic potential there is a dire need to develop cheap recombinant Hsp70 production and further investigate the interaction of externally supplied and endogenous Hsp70 in chaperonotherapy.
Ritossa’s description of morphological changes in the structure of Drosophila polytene chromosomes (formation of puffs) after heat shock (HS) opened a new page in the study of inducible genes and the entire eukaryotic genome [1]. Interestingly, this pioneering work was not immediately appreciated and was first regarded as a kind of amusing mishap, characteristic only for Drosophila. It was not until the mid-seventies, when the molecular consequences of HS were discovered [2, 3], that an avalanche of studies began on this system, which appeared to be present in the genomes of all organisms studied [4, 5, 6, 7, 8, 9]. Its simplicity and high reproducibility have made the HS gene system very popular for studying the subtle mechanisms of eukaryotic gene regulation and genome function in general [10, 11, 12, 13, 14, 15, 16, 17, 18].
A comprehensive study of the HS gene system carried out on different objects from bacteria and flies to humans has shown the presence of different groups of HS genes and demonstrated their role in cell and organism functioning in general. Excellent reviews are describing the structure and function of different classes of HS genes [19, 20, 21, 22, 23].
In particular, the role of different classes of Hsp and especially Hsp70 in the maintenance of cellular proteostasis, both under normal physiological conditions and after stress, has been demonstrated in different laboratories [24, 25, 26, 27, 28]. It was also shown that the Hsp70 protein plays the central role in the folding and assembly of newly synthesized proteins, refolding of misfolded and aggregated proteins, membrane translocation of organelle and secretory proteins, and control of the activity of various regulatory proteins [29]. On the other hand, field biologists, as is often the case, did not immediately pay attention to the hsp gene system, and started to study these genes in an ecological aspect later [30, 31, 32, 33, 34, 35].
It was logical to assume that if Hsps are really so important for the normal functioning of the cell and the organism as a whole under normal and stressful conditions, the hsp gene systems in organisms living in arid climatic zones or areas with rapid temperature fluctuations should characteristically differ from similar systems of forms from temperate climate zones. In fact, it turned out to be so. Several examples have shown that thermophilic species are capable of synthesizing Hsps at higher temperatures compared to phylogenetically similar species from temperate zones [7, 30, 36, 37].
In recent decades, molecular ecology has accumulated numerous facts and observations indicating the important role of various Hsps and especially the main stress protein Hsp70 for the adaptation of organisms to adverse conditions, as well as describing how the structure of hsp genes and correspondent proteins evolved for their optimal functioning under various conditions, including extreme ones [7, 38, 39]. Heat shock genes play an important and sometimes even pivotal role in adaptation to changing environmental conditions not only in eukaryotes, but also in bacteria, especially in pathogens and symbionts [8].
Although we are aware of the role of other Hsp groups in adaptation to various stress conditions [40, 41, 42, 43, 44], in this review we will pay special attention to the role of the major stress protein (Hsp70) in adaptation to adverse conditions. Besides, herein we consider the examples of the use of recombinant Hsp70 for the treatment of various diseases and pathologies in both model animals and humans available in the literature.
It was established that the rapid activation of all groups of hsp genes under stress at the transcriptional level is provided by the same protein factor (Heat Shock Factor-HSF1). All hsp genes contain heat shock elements (HSE) consisting of several inverted repeats of the consensus nGAAn sequence in the proximal part of the promoter region [45, 46]. In the absence of stress, HSF1 monomers interact with Hsp70 and Hsp90 to form an inactive complex. During heat shock, an increase in the number of denatured, unfolded proteins in the cell causes decay of the Hsps and HSF1 complex, and Hsps bind to the damaged proteins. It should be mentioned that activation of HSF1 involves several steps, including extensive posttranslational modification, translocation into the nucleus, trimerization, and binding to heat shock elements (HSE) at promoter sites, inducing transcription of all HS genes [47, 48, 49].
To date, it has been shown that the regulation of Hsps expression in particular is more complex and may differ in different tissues and organs [49, 50, 51]. The search for tissue-specific regulators of HS gene induction is important for understanding the role of Hsps in adaptation as well as in the treatment of many diseases, especially neurodegenerative ones.
An important feature of genes belonging to the hsp70 family is their multi-copy nature. Indeed, in the vast majority of the studied animal species, hsp70 genes are represented by several copies. Thus, in Drosophila, there are 5 to 6 copies of genes of this family induced under the action of HS [52, 53]. Thirteen genes of the hsp70 family were found in the human genome [54, 55], and several of them are expressed constitutively, sometimes only in certain tissues, while other members are induced under various stress influences [54, 56]. It is also important to note the unusually high homology between genes encoding hsp70 in different organisms. Thus, the homology between Drosophila and human hsp70 genes reaches 72%, and between human and E. coli hsp70 genes – 50% [57]. Interestingly, in Drosophila the basic structure of hsp70 genes is a pair of genes in an inverted position, and in thermophilic Drosophila species, the number of hsp70 genes tends to be higher than in species from temperate zones [7, 52, 58]. Another important and obviously adaptive feature of hsp70 genes is their cluster organization. It turned out that in thermophilic species these clusters have a more compact structure, which apparently provides their cumulative effect under stress. It was also shown that the high homology of the hsp70 gene copies included in such a cluster is maintained by gene conversion [7, 59, 60].
Characteristic features have been described for hsp70 genes at the chromatin level as well. Thus, back in the 1980s, it was shown that the regulatory regions of the Drosophila hsp70 genes are represented by “naked” DNA, that is, devoid of nucleosomal structure [61, 62, 63]. Characteristically, the promoters of hsp genes under normal temperature are associated with a suspended, positioned RNA polymerase II (RNAPII) [64]. Such promoter arrangement is obviously necessary for rapid and efficient turn-on of all hsp70 genes under stress. Interestingly, naked DNA regions in hsp70 genes are hot spots for the insertion of mobile elements both in the case of P-mutagenesis and spontaneous transposition of mobile elements, which apparently ensures rapid evolution of these regulatory regions and hsp70 genes in general [65, 66]. At this end, in an in vitro system, it has been shown that hsp70 gene promoters of the camel, whose cells are significantly more heat tolerant compared to human cells [32, 67], have higher “strength” at elevated temperatures compared to orthologous human hsp70 promoters [68].
Studies of hsp70 gene expression in different organisms from temperature-contrasting climatic zones have allowed to describe characteristic features of this system functioning under normal physiological conditions and stress [7, 34, 37, 39, 69, 70, 71, 72, 73]. In this regard, we should recall the pioneering work of Ulmasov et al. [71] who showed that the level of Hsp70 in the body of desert, temperature-resistant lizards changes characteristically depending on the ambient temperature during the day. Adaptive changes in the level of Hsp70 expression depending on the season were also shown in other studies [74, 75], indicating the ecological importance of Hsps synthesis in natural populations. It should be emphasized that constitutive expression of inducible Hsp70 genes in different species from arid zones or regions with sharply changing temperatures during the day is probably the main feature characteristic for such species. This phenomenon has been described for such different groups of animals as lizards, ants, flies, tidal zone dwellers, Baikal amphipods, etc. [7, 32, 33, 34, 37, 39, 69, 70, 71, 72, 73].
The constitutive expression of Hsp70 and other chaperones in the cells of thermoresistant species apparently allows such forms to maintain proteostasis and function normally under high temperatures without switching on additional adaptogenic systems. An alternative system of regulation of hsp70 genes has usually been observed in temperate forms, that are rarely exposed to sharp temperature fluctuations. In such species, of which Drosophila is a typical example, under normal temperature conditions Hsp70 synthesis is at a low, difficult-to-detect level, but when temperature or other stress influences are increased, rapid and intense activation of all hsp genes and, in particular, of the hsp70 gene battery is observed [76]. Rapid and intense induction of Hsp70 in Drosophila species apparently determines their ability to acclimatize. Drosophila species inhabiting habitats with higher temperatures are characterized by a larger number of hsp70 genes, a compact organization of their clusters, “stronger” promoters, and, consequently, more powerful induction of Hsp70 with increasing temperature, compared to species inhabiting temperate habitats [58, 77, 78]. It should be noted that a low, basal level of Hsp70 observed in Drosophila species is still, apparently, necessary for the normal functioning of flies under non-extreme physiological conditions. Thus, it has been shown that D. melanogaster flies with deletion of all copies of hsp70 genes have impaired survival after severe HS, as well as a tendency to develop various neurodegenerative processes [79]. It has also been demonstrated that males of this strain with deleted hsp70 genes have completely impaired memory and learning ability; moreover, low irradiation resulted in reduced viability in flies lacking hsp70 genes [80, 81]. It should be noted, that the observed patterns of Hsp70 synthesis of the “Drosophila type” characterized by the low constitutive synthesis of Hsp70 under normal physiological conditions and the “Lizard and Ant type”, when a pronounced constitutive synthesis of Hsp70 is observed in the cells of arid zone animals, by no means exhaust the spectrum of eukaryotic genome response to extreme environmental conditions. Several examples from different animal groups were described that do not fit into this simple scheme [82, 83, 84, 85]. For example, it was shown that selected D. melanogaster flies capable of living and reproducing at 31 °C are characterized by lower Hsp70 induction during HS compared to other strains of this species [86, 87]. Cold-living (e.g., Antarctic) species that dwelled for millions of years under stable low-temperature conditions and completely lost the ability to induce hsp genes when temperature rises have also been described [83]. Such forms are characterized by a loss of canonical HSEs within hsps promoters [88] (Fig. 1A).
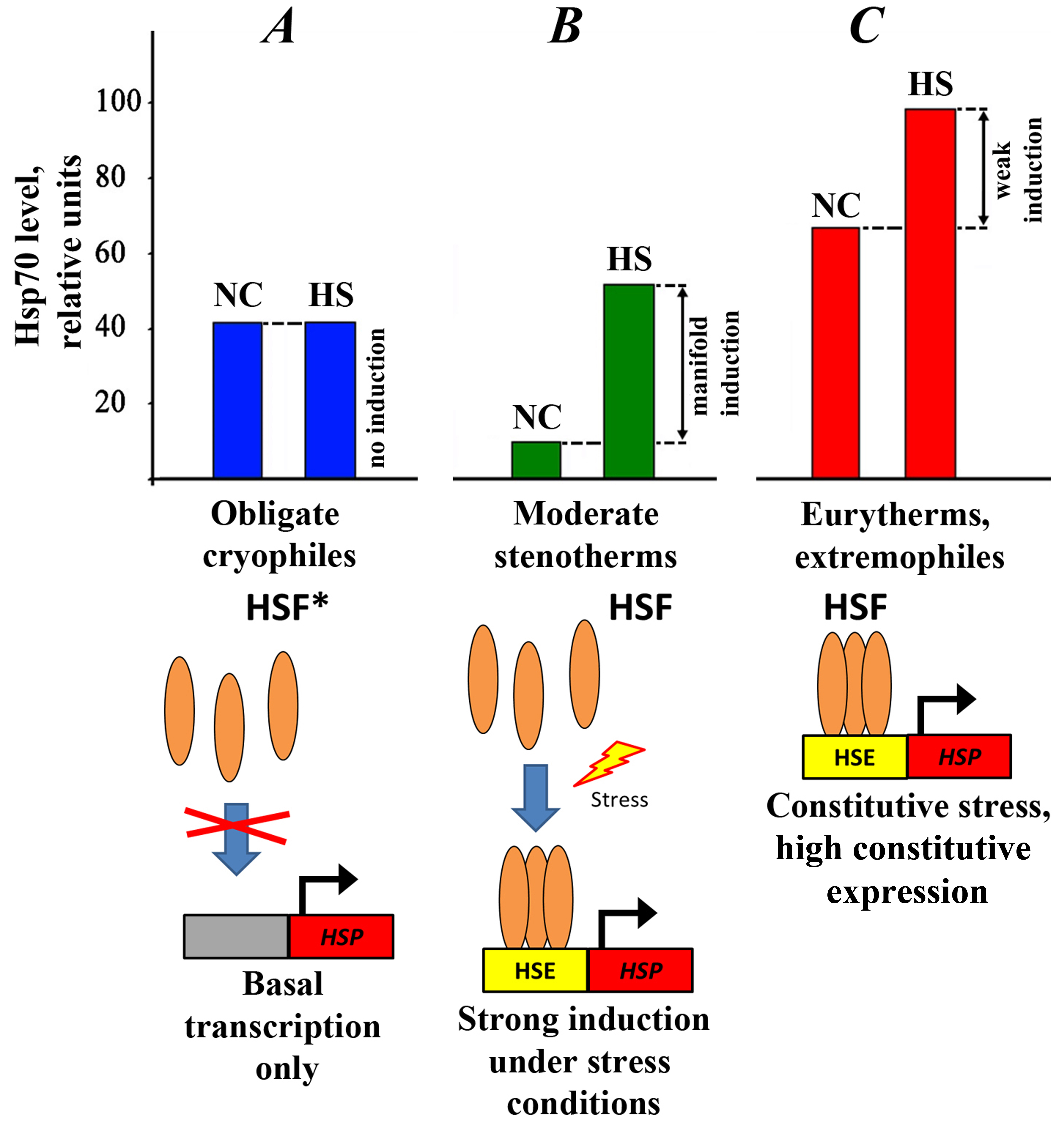
Peculiarities of heat shock response regulation in organisms that dwell in temperature contrasting habitats. (A) Cold-living (obligate cryophiles) Antarctic forms dwelling in stable low-temperature conditions lost the ability to induce hsp genes when the temperature rises due to loss of HSE sequences within hsp genes promoters. (B) Species living in a temperate climate zone with rare sharp temperature fluctuations normally synthesize low levels of Hsps, that are dramatically induced after HS. (C) Extremophiles, thermoresistant species living in hot climates and constitutively synthesize high levels of Hsp70, which increases slightly after HS. HSF* - in some species from the group of obligate cryophiles, the ability to activate HSF is lost due to mutations in the hsf gene.
It should be noted that the hsp70 genes system present in all eukaryotic organisms is a complex, well-tuned adaptive mechanism that underwent millions of years of evolution, and any experimental manipulation with this balanced system can lead to unpredictable consequences. For example, transgenic Drosophila strains with the experimentally increased number of hsp70 copies have reduced viability and other developmental disorders [89].
It is of note that heat shock genes play essential roles in adaptation to extreme environmental conditions in bacteria as well, particularly in pathogens and symbionts. In bacteria, Hsp70 proteins contribute to their survival in a hostile environment. It is noteworthy that the adhesion of bacteria to host cells is mediated by both host and bacterial Hsp70 (DnaK). After infecting the host, it is DnaK that initiates the processes of bacterial survival and induces the host’s immune response. Any mutation in the DnaK gene reduces the viability of bacteria in the host [9].
Convergent evolution resulted in more similar amino acid sequences in inducible Hsp70 in different heat-tolerant, phylogenetically distant species compared to evolutionarily close forms living in milder climates [90, 91]. Probably, in this case, natural selection acted at the amino acid level, selecting variants of Hsp70 capable of providing maximum protection under extreme temperatures and other forms of stress. To test this assumption, we introduced into the genome of D. melanogaster with deleted all hsp70 genes a copy of the orthologous gene isolated from a Stratiomyidae (Diptera) species whose larvae live in hot, sulfurous springs of the Kuril Islands and can withstand temperatures up to 42–45 °C [70]. Our experiments showed that the larvae of such a transgenic strain containing a single hsp70 gene isolated from the genome of this highly thermostable species can withstand higher temperatures compared to the D. melanogaster larvae containing one copy of its own endogenous hsp70 gene [92]. Characteristically, experiments using in vitro refolding luciferase assay demonstrated that Stratiomyidae Hsp70 exhibited higher refolding capacity in comparison with D. melanogaster Hsp70 and even human paralog [91]. Moreover, using differential scanning calorimetry we showed that the ATP-binding domain of Stratiomyidae singularior Hsp70 is stable at temperature 4 degrees higher than that of Drosophila Hsp70 [91]. It is of note, that two isoforms of inducible Hsp70 were detected in exceptionally thermoresistant arid zone lizards, and the synthesis of each of these forms occurred at different elevated temperatures [71]. Thus, many researchers have shown using various objects the protective, chaperone role of Hsp70 under various extreme conditions, as well as the participation of Hsp70 in the maintenance of cellular proteostasis, both under normal and stressful conditions.
Generally speaking, Hsp70 plays an exceptionally important role in cell membrane function [93]. As early as 1986, Hightower suggested that Hsp70 might associate directly with plasma membrane lipids [94, 95]. It was later demonstrated, that Hsp70 plays an important role in whole organism protection under stress through the maintenance of barrier functions of endothelium and epithelium [96]. It is known that with elevated body temperature a general intoxication of the body usually occurred associated with the release of toxins and bacteria (e.g., endotoxins, lipopolysaccharides) from the gut, as well as an increase in circulating cytokines in response to the toxins. This is associated with an increase in the permeability of the intestinal epithelium during intense exercise and heat stress [97, 98, 99]. Increased expression of Hsp70 has been shown to prevent heat shock-induced breakdown of the epithelial barrier by stabilizing tight junctions between epithelial cells and improving its recovery from HS and other forms of stress [100, 101, 102]. The role of Hsp70 in resistance to heat-induced damage in humans was demonstrated in a study of Kuennen et al. [103], which showed that inhibition of the HS response by Hsp70 inhibitor quercetin during 7 days of heat acclimatization resulted in a significant increase in gastrointestinal permeability and endotoxin leakage after acute heat stress.
In addition to protecting the intestinal epithelium from stressors, Hsp70 plays an important role in maintaining the integrity of the vascular epithelium [104, 105]. More recently Hsp70 has been shown to interact selectively with negatively charged phospholipids, particularly phosphatidylserine (PS) [106, 107, 108, 109, 110] inside liposomes, after which Hsp70 is incorporated into the lipid bilayer, forming high molecular weight oligomers [107] that have ionic conduction channel properties [111, 112].
Besides, phosphatidylserine in membranes, Hsp70 interacts with several other negatively charged phospholipids, most notably bis(monoacylglycero)phosphate (BMP), the main phospholipid of lysosome membranes [113, 114]. The binding of Hsp70 in lysosomal membranes to the negatively charged phospholipid BMP imparts stability to this compartment by inhibiting lysosomal membrane permeabilization and preventing the release of lysosomal proteases and cathepsins into the cytosol in response to stressful conditions, thereby preventing cells and organ death [113, 115].
There is extensive evidence that Hsp70 stabilizes biological membranes [116, 117, 118]. Thus, the temporal association of Hsps with membranes can restore bilayer fluidity and thus maintain membrane functionality under stress [116]. The accumulated data show that Hsp70 is preferentially embedded in cholesterol-rich microdomains (“rafts”) that serve as major platforms for the assembly and sorting of signal transduction complexes in the membranes (Fig. 2) [119]. It has been also demonstrated that Hsp70 can play a role in maintaining the stability of lipid raft-associated signal transduction complexes after stress [120]. Moreover, after various stresses and in some forms of cancer, free Hsp70 accumulated in the cell can be incorporated into the plasma membrane and be further released into the extracellular space both in free form and in the form of lipid vesicles of exosomes [121], lysosomal endosomes [122] or the context of cholesterol-rich microdomain-rafts (Fig. 2) [123].
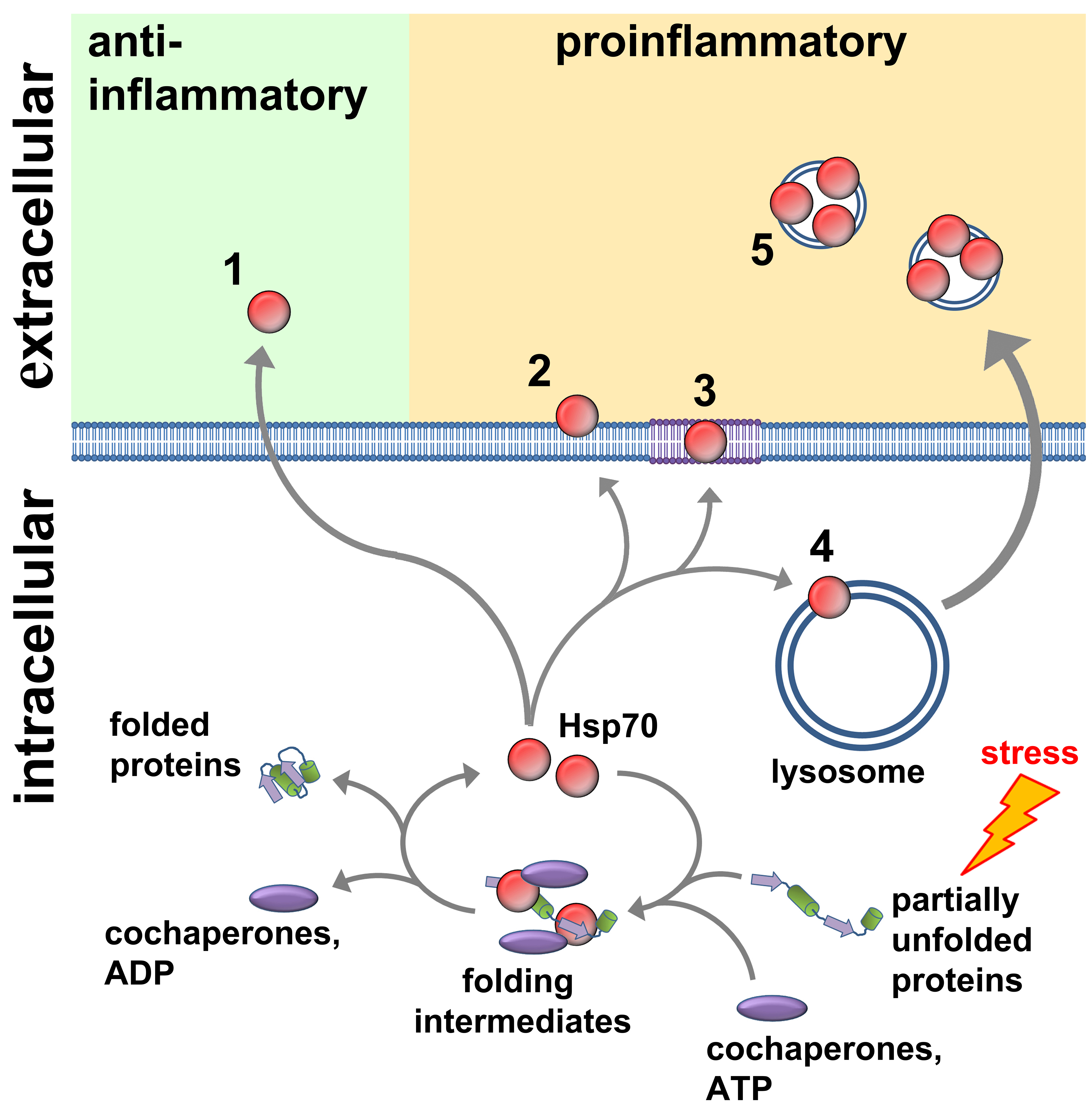
Intracellular Hsp70 functions as a molecular chaperone, preventing aggregation and restoring native conformation of partially unfolded proteins with the participation of ATP and several co-chaperones. Extracellular Hsp70 may exist in two different forms, free (1) and membrane-bound (2–5). (1) Free protein releases from cells after necrosis under the action of various damaging factors, or due to transmembrane transport by a non-classical mechanism that does not require a signal sequence, involving the ABC transporter. (2, 3) Membrane-bound Hsp70 comes to the surface of the cell by interaction with phosphatidylserine as a part of the inner lipid layer of the membrane and subsequent transfers together with the phosphatidylserine molecule into the outer layer and lipid rafts. (4, 5) a result of secretion of Hsp70 as a part of membrane vesicles (ectosomes and exosomes), Free, unbound to membrane structures Hsp70 and exogenous recHsp70 predominantly play an anti-inflammatory role, while membrane-bound Hsp70 may play a pro-inflammatory role. By binding to the membranes of lysosomes, Hsp70 prevents their permeabilization and release of lysosomal secretion into the cell.
It has been also shown, that extracellular Hsp70 (eHsp70) interacts with several receptors on the cell surface and may be internalized by target cells and initiate various signaling cascades. Thus, several receptors such as TLR2/4, CD91, CD40, and SR appear to be responsible for eHsp70 phagocytosis [124, 125].
The unique protective characteristics described for Hsp70 in numerous in vivo and in vitro studies served as a basis for the investigation of the involvement of this protein in various diseases and its possible therapeutic potential.
Studies of the role of Hsps, and Hsp70 in particular, under normal physiological conditions and after the stress has demonstrated the crucial role of this system in proteostasis at different steps of protein synthesis and degradation [5, 7, 24, 126, 127]. In substrate degradation, Hsp70 explores the ubiquitin-proteasome system as well as various autophagy pathways [27]. Naturally, it was interesting to find out how the Hsp70 family members function in the case of various pathologies accompanied by disturbances in cellular protein synthesis and degradation, and whether the levels of Hsp70 and other chaperones in various tissues can be used as an indicator of disease and physical condition in humans. Indeed, it was shown that during normal aging, the level of Hsp70 is significantly reduced in animals and humans [128, 129, 130]. Hsp70 levels decrease in humans during aging in most organs, including neurons, leading to the accumulation of damaged and unfolded proteins. This contributes to proteotoxicity, which may lead to the development of age-related diseases due to neurodegeneration. Aging also leads to the attenuation or alteration of many signaling pathways, as well as the expression of several transcription factors, including major heat shock factor (HSF1) [129, 130, 131]. Characteristically, aging decreases the level of Hsp70 induction after stress [132]. A decrease in the expression level of Hsp70 regardless of age is observed in such human diseases as pulmonary fibrosis, various types of “myopathies”, cholesterol sphingolipids, sphingolipidoses, diabetes, obesity, etc. [133, 134, 135, 136, 137]. In these diseases, reduced levels of both HSF1 and various members of the Hsp70 family can be observed in adipose tissue, liver, muscle, neurons, and vascular beds of patients. Interestingly, different baseline, constitutive levels of various chaperones, and particularly Hsp70, detected in patients often determine the severity of the disease course and the outcome of various diseases, including atherosclerosis and COVID-19 [138, 139]. Therefore, the level of endogenous Hsp70 in cells is used, not only as a biomarker of various diseases but also to monitor the results of therapy for various human diseases [139, 140]. In particular, Hsp70 levels have been used as a biomarker of prostate diseases and bronchopulmonary dysplasia (BPD) for targeted therapies [135, 141]. Thus, it is known that Hsp70 levels are associated with a state of oxidative injury in the development of BPD [135]. Significant changes in Hsp70 levels in neuronal tissues have been reported in normal aging as well as in patients with neurodegenerative diseases accompanied by the formation of protein aggregates, including Alzheimer disease (AD), Parkinsonism, and amyotrophic lateral sclerosis (ALS) [142, 143, 144]. Determination of the Hsp70 levels and some other chaperones can serve as an indicator of the development of pathology in the case of AD. However, changes in Hsp70 levels in various pathologies may be multidirectional for members of the Hsp70 family in different brain tissues of the patients. Thus, in AD, HSPA8 levels are increased in brain cells, while HSPA1A and HSPA2 levels are decreased [145]. This may be explained by the fact that members of the Hsp70 family are not equally expressed in different human tissues under normal conditions [54].
It is known that a significant increase in Hsp70 levels is often observed on the surface of cancer cells of different origin [143, 146], which may hinder anti-cancer therapy. Thus, the presence of Hsp70 on the surface of cancer cells and its interaction with lysosome membranes have been envisioned as potential therapeutic targets [146, 147]. Generally speaking, Hsp70 membrane association is a key component in the extracellular export of these proteins [148]. The secreted Hsp70 can additionally act as an effective neuroprotector, increasing the survival of neurons in various proteinopathies, as has been demonstrated in Alzheimer’s and Parkinson’s disease models. In this regard, recHsp70 and inducers of endogenous Hsp70 synthesis may be considered as candidate therapeutics with immune-modulating and neuroprotective properties. Since various members of the Hsp70 family, are predominantly expressed in different tissues and organs [54], it should be taken into account when using Hsp70 as an indicator of pathology and when performing therapies aimed at normalization or increase of Hsp70 levels in a particular tissue in various diseases. The changes in the levels of various Hsp70 family members in various diseases and pathologies demonstrated by many researchers served as a basis for conducting experiments on correction of Hsp70 levels in model animal and human cells both by induction or suppression of endogenous Hsp70 synthesis and by introduction of exogenous recHsp70 by various means.
A variety of experimental approaches has been used to normalize the level of endogenous Hsp70 impaired in various pathologies, including aging and chronic diseases. In some cases, researchers activated the synthesis of all Hsps groups, i.e., increased the heat shock response (HSR) in general, for example, by increasing the temperature of the body and proved, for example, the role of HS-induced Hsp70 for muscle regeneration in a mouse model [149]. Sometimes, simultaneous change of the expression level of all members of the Hsp70 family and other chaperones has been achieved by regulation of the main heat shock transcription factor (HSF1) synthesis [150]. In several other cases, the investigators have succeeded in selectively increasing the synthesis of only Hsp70. Such an approach has been recently applied to suppress a cytokine storm in COVID-19 patients [151]. Sometimes researchers used a combined approach, inducing HSR and administering recHsp70, for example, to treat diseases such as diabetes mellitus [137]. It is noteworthy to mention in this regard the results of Lydia Kitchen, who used photobiomodulation therapy (irradiation by 1060–1080 nm light), a noninvasive method of modulating Hsp70 and nitric oxide (NO) levels to treat infection in the case of COVID-19 and other coronaviruses [152], and the application of selective Hsp70 induction in the treatment of ultra-rare neuromuscular disorder GNE myopathy [134]. Along these lines, a specific low molecular weight inducer of Hsp70 (“KD-23”) exhibited a therapeutic effect in a cellular model of craniocerebral injury [153]. Similarly, selective induction of endogenous Hsp70 with dioscin or geranylgeranylacetone (GGA) has been successfully applied in various lung injuries including fibrosis, and to restore blood flow after ischemia [154, 155].
A separate group of naturally occurring substances capable of increasing the level of Hsp70 and other chaperones in the cell is represented by the so-called “adaptogens”, i.e., natural substances such as ginseng, Eleutherococcus, radiola rosea, etc. These substances are usually of plant origin, and their use is often accompanied by induction of Hsp70 in various human tissues and organs [156, 157, 158, 159]. Such substances have long been successfully used in China and other countries to heal wounds, as well as in several chronic and aging-related human diseases. The successful use of inducers of the entire HSR system as well as experimental selective modulation of the level of endogenous Hsp70 in the treatment of various diseases showed the promise of this major stress protein as a therapeutic agent and provided the basis for the production and use of recHsp70 in medicine.
Various laboratories have developed various methods for the expression and isolation of recHsp70. RecHsp70 has been isolated from a variety of animal and plant objects, produced in bacteria, and expressed in various cell cultures, including human cells, as well as in the milk of transgenic animals [139, 160, 161, 162, 163]. The use of recHsp70 has shown its efficacy in many models of human diseases with a variety of administration methods, from intranasal and intravenous to intracranial [161, 164, 165].
Thus, preventive intravenous administration of human recHsp70 in a rat model of sepsis normalized lipopolysaccharide (LPS)-induced blood pressure abnormalities and biochemical blood parameters and significantly reduced animal mortality [166]. Similar results were obtained with intravenous administration of a single dose of recHsp70 in an LPS-induced mouse peritonitis model [167].
In a mouse model of aging, we were able to show that intranasal subchronic administration of recombinant human Hsp70 significantly extends animal life and had a profound rejuvenation effect in neuronal cells of the cortex and hippocampus. Interestingly, an increase in lifespan when Hsp70 was sub-chronically injected was observed only in males [164]. In the mouse aging model used, as well as in two validated AD models, it was demonstrated that subchronic intranasal administration of recHsp70 improved memory and learning ability and reduced the level of such markers of aging as lipofuscin and amyloid level [164, 168].
The method of intranasal administration of human recHsp70 used also gave encouraging results in a model of photothrombotic stroke in mice [169]. Similarly, in a mouse model of diabetes, researchers were able to increase insulin sensitivity with intranasal injections of cheap Hsp70 isolated from alfalfa [161]. Kirkegaard et al. [162] first applied recHsp70 and its inducer to treat various forms of lysosome storage diseases, using both various animal models and cell cultures of patients, with various disorders of lysosome biogenesis (aggregation).
Administration of recHsp70 in different ways led to positive therapeutic effects when used in vitro and animal models for such different diseases and pathologies as diabetes, prostatitis, and various forms of lung damage, including various forms of fibrosis [135, 137, 141, 170]. RecHsp70 administration also resulted in a reduction of cholesterol levels in human cells and model animals [171]. In this context, the exploration of the lentiviral constructs to express Hsp70 in different tissues in the case of lung injury should be mentioned [172]. It’s of note, however, that in various forms of fibrosis, viral infections including COVID-19 cases, as well as in different forms of cancer, the administration of recombinant Hsp70 or its experimental induction may have different, sometimes multidirectional effects depending on the disease, dose of the chaperone and time of administration [140, 146, 147]. Thus, on the one hand, the increased content of endogenous Hsp70 on the cell membrane observed in many forms of cancer can hinder successful anti-cancer therapy, on the other hand, administration of recHsp70 in Margulis’ laboratory and several other groups had a significant therapeutic effect both in vitro when used on various cancer cells and in vivo in pilot experiments [173, 174, 175, 176], where injections of recombinant human Hsp70 were made directly into tumors of volunteers [176]. In their study of melanoma cells, this group showed that injection of recombinant Hsp70 triggers extracellular transport of its endogenous homolog in soluble form and as EVs (extracellular vesicles), that penetrate neighboring cells, resulting in a dramatic reduction in the growth rate of cancer cells [177]. In another study on carcinoma cases, the patients were injected with dendritic cells containing hsp70 mRNA induced by electroporation, and a positive effect was also observed in several patients [178].
Although large-scale studies on the role of Hsps and especially Hsp70 in various diseases, including cancer, as well as in normal aging, are currently underway in various laboratories, there are only a relatively small number of researchers taking practical steps to apply recombinant Hsp70 in the clinic. This is due, firstly, to the high cost and low availability of the recombinant human Hsp70 preparation and, most importantly, to the insufficient data and analysis of the consequences of Hsp70 application in patients, given the variety of interactions of Hsp70 with different proteins and cellular signaling systems.
Intensive research on the role of endogenous and recombinant Hsp70 in aging, as well as in a variety of diseases and pathologies in model animals and humans resulted in the description of a variety of factors and signaling pathways with which Hsp70 interacts in carrying out its protective functions. Numerous excellent reviews are describing the functions of Hsp70 under normal conditions and after stress [5, 24, 126, 141, 144, 177]. Therefore, in this section, we will only briefly discuss the known properties of Hsp70 that can be used in the therapy of some human diseases.
It should be noted that generally speaking, Hsp70 has two main functions in the body. First, members of this family together with Hsp90 and co-chaperones (e.g., Hsp70-Hsp90 Organizing Protein (HOP)) provide proteostasis in cells under normal conditions and stress [7, 24, 126, 127]. Secondly, it was shown that Hsp70 can excrete from many types of cells into the intercellular medium and interact with several receptors on the surface of cells responsible for innate immunity (specially TLR2/4 receptors). Initially, results indicated that extracellular Hsp70 (eHsp70) causes the activation of macrophages and neutrophils and the production of pro-inflammatory cytokines. Based on these data, it has been suggested that Hsp70 represents a “danger signal”, activating the immune system and thus playing a regulatory “cytokine” role therefore Hsp70 is often called “chaperokine” [179].
However, later it was shown that these pro-inflammatory effects are often caused by the action of residual amounts of LPS, with which recombinant Hsp70 preparations used in these works obtained in E. coli were contaminated. Subsequently, it was shown that free extracellular Hsp70 obtained in eukaryotic expression systems has rather an anti-inflammatory effect. In inflammatory processes occurring during various diseases, both the induction of endogenous Hsp70 and the administration of LPS-free recHsp70 reduce the levels of proinflammatory cytokines (TNF-a, etc.), diminish reactive oxygen species (ROS) and NO content, and restore cellular redox status [130, 140, 151, 155, 180]. It was further shown that different forms of exogenous Hsp70, in particular, free and membrane-bound ones, can have the opposite effect on the activity of the immune system cells (see below).
The ability of Hsp70 to bind to a variety of receptors and compete with other ligands plays a major role in several cases. An example of such interactions is the anti-inflammatory role of recHsp70 described in several works in LPS-induced sepsis, where recombinant Hsp70 competes with LPS for binding to TLR-4, which leads to restoration of basic blood parameters and increased animal survival [166, 167].
Interestingly, in our experiments administration of recHsp70 significantly reduced LPS-induced TLR4 expression in human macrophage cells (THP1) [181].
The ability to eliminate damaged proteins and prevent the formation of
aggregates of different nature is obviously the most important protective
function of Hsp70 described for such diseases as AD, Parkinsonism, ALS, and many
others. It was shown that recHsp70 can compensate for the insufficient level of
endogenous Hsp70 often observed in these pathologies [143, 144]. Thus, in various
models of AD, the intranasal administration of recHsp70 reduces
It is also worth mentioning an important and well-described role of Hsp70, which manifests itself as an anti-apoptotic agent at the early stages of this process [183, 184]. This explains its crucial role in such diseases as cancer, pulmonary fibrosis, ischemia, neurodegeneration, etc. Thus, an investigation of the role of Hsp70 in lung dysfunction of model animals as well as in LPS-treated human alveolar epithelial cells showed that Hsp70 interacted with KANK2 (ankyrin-repeat domain-containing protein), leading to reversed cell viability and reduced level of apoptosis-inducing factor (AIF) and, hence, apoptosis [172].
On the other hand, as we pointed out earlier, in some forms of cancer these anti-apoptotic properties of Hsp70 may interfere with anticancer therapy, forcing the use of Hsp70 inhibitors in these cases [185, 186].
Hsp70 plays an important role in various viral diseases, including COVID-19.
Here, as well as in the case of cancer cells Hsp70 can play a dual role being “a
double agent”. On the one hand, endogenous Hsp70 binding to TLR4 receptors may
interfere with virus penetration into the cell. In addition, Hsp70 can prevent
cytokine storm, which is the main cause of death in COVID patients, by causing
degradation of the p65 subunit of the nuclear factor kappa-light-chain-enhancer
of activated B cells (NF-
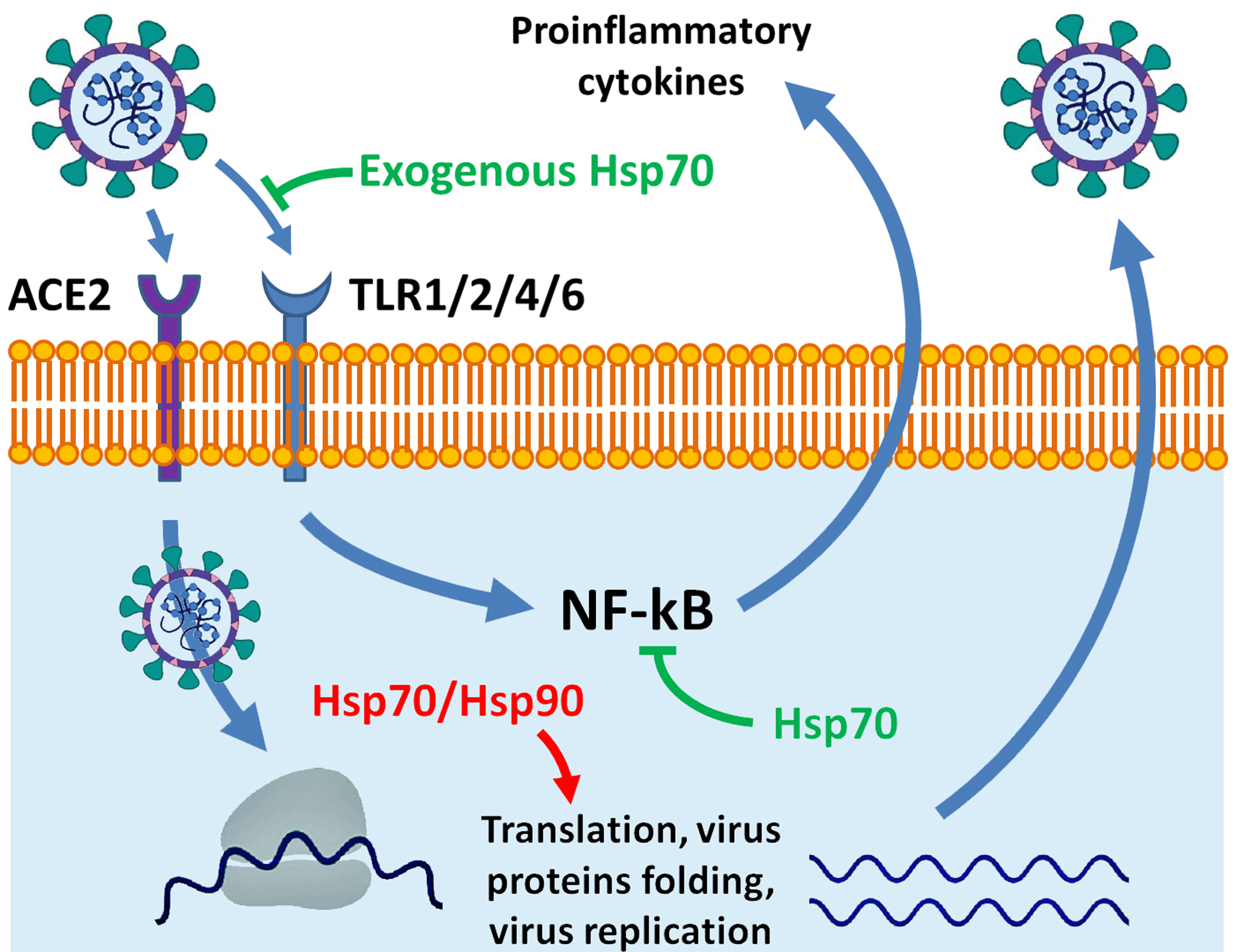
In viral infection (SARS-CoV-2), extracellular HSP70, by binding to TLRs
and probably to ACE2 receptors, blocks them and prevents the penetration of the
virus inside the cell. Hsp70 also blocks NF-
As numerous studies demonstrated Hsp70 family members are highly effective vaccine adjuvants [188, 189, 190]. Thus, for example, the increase in the effectiveness of vaccination with laser irradiation of the skin in the area of influenza vaccine administration is associated with the induction of endogenous Hsp70, which has the properties of a vaccine adjuvant [191].
One of the most important protective functions of Hsp70 in many diseases is due to its role as an immunomodulator [192]. In particular, in our experiments exploring a mouse AD model, we showed that chronic Hsp70 administration changes the expression of several genes in the hippocampus of model animals. Most importantly using RNA-Seq, we identified a lot of differentially expressed genes in the hippocampus of old Tg mice compared with those of non-transgenic mice of the same age. Specifically, we observed Hsp70-induced upregulation of multiple genes participating in antigen processing and presentation especially the members of major histocompatibility complex (class I and II) in the brains of old 5XFAD Tg animals, suggesting that Hsp70 executes its beneficial role via activation of adaptive immunity [193].
In a model of infectious lung inflammation, it was shown that intraperitoneal injection of recHsp70 reduced the number of programmed death-1 (PD-1) positive T-lymphocytes in peripheral blood by several times and improved other important lung characteristics such as lung coefficient (Fig. 4) [181].
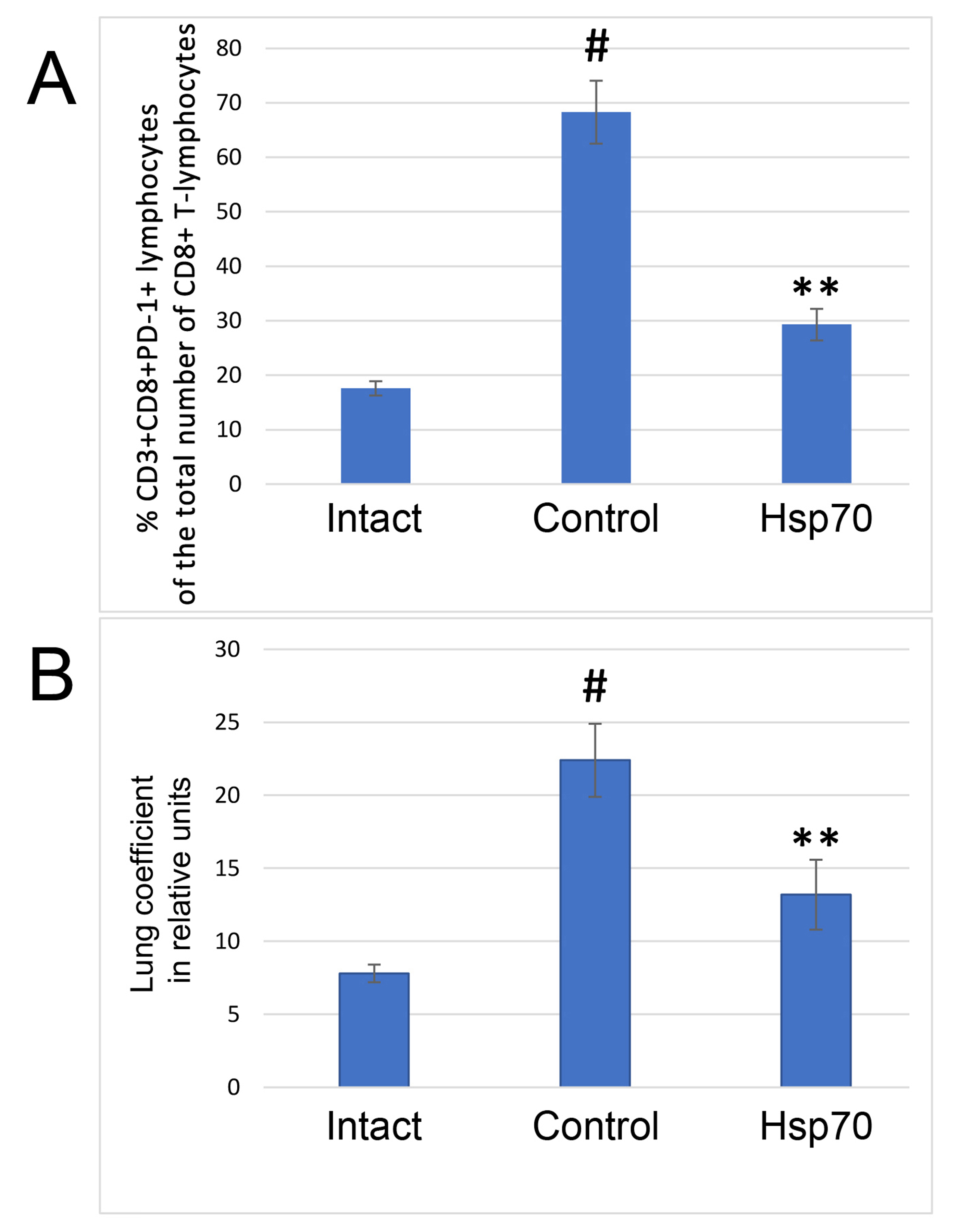
Protective effect of Hsp70 in mouse the pneumonia model. RecHsp70
preparations decreased level of T lymphocytes with inhibitory PD-1 receptors in
peripheral blood (A); and pulmonary coefficient (B) in the case of
influenza-induced pneumonia in mice. # p
In a study of melanoma cells (B16), the addition of recHsp70 caused active synthesis and release of the endogenous analog from the cells in soluble and EVs forms, and both forms actively penetrated neighboring cells, leading to a sharp increase in Natural Killer (NK) cell toxicity towards melanoma cells [174]. This immunomodulatory effect of Hsp70 was due to the enhanced CD-8 positive response and massive anti-tumor cytokine accumulation.
In addition, numerous signaling systems and specific factors with which Hsp70 interacts in various diseases have been described in recent years. Thus, the interaction of Hsp70 with the androgen receptor (AR) and the important role of this chaperone in the pathogenesis of prostate cancer has been shown [141].
Another interesting example of the regulatory role of Hsp70 is the demonstrated ability of recHsp70 to lower cholesterol levels in primary human macrophage foam cells. Thus, RNA-seq analysis showed that the addition of Hsp70 led to the reprogramming of cell expression, including up-regulation of key targets of liver X receptors (LXR), master-regulators of whole-body cholesterol removal [171].Valeria Calvaresi’s group using native and mutant forms of recHsp70 described the details of Hsp70 binding to lysosomal membranes, which protects a whole series of lysosomal storage diseases [194].
It should also be noted that members of the Hsp70 family actively interact with
other adaptive systems of the body. In particular, they interact with the system
of genes responsible for the synthesis and metabolism of hydrogen sulfide
(H
Along these lines, in our experiments on a small sample of Covid patients [181], we have recently demonstrated that hot helium inhalation inducing Hsp70 followed by inhalation of hydrogen sulfide donor has a pronounced therapeutic effect preventing lung fibrosis in the patients. Moreover, in our in vitro and in vivo experiments we showed that different hydrogen sulfide donors as well as recHsp70 significantly reduced the level of proinflammatory cytokines, ROS, and other consequences of LPS-induced inflammation [181]. The contradictory and difficult-to-explain results sometimes obtained when analyzing the action of endogenous and recHsp70 may be related to the fact that different members of this family are preferentially synthesized in different organs and tissues and to the numerous tissue-specific posttranslational modifications to which members of the Hsp70 family are often subjected [180, 196].
The discovery of the HS-inducible genes by Ferruccio Ritossa back in the early 1960s opened a new page in the study of the molecular mechanisms controlling the expression of the eukaryotic genome. While the main efforts of scientists were aimed at studying the structure and molecular regulation of hsp genes, the role of these genes and, in particular, the role of the main stress protein Hsp70 in adaptation to extreme environmental conditions attracted the attention of ecologists and molecular biologists much later. In the course of such studies, it was shown in a wide spectrum of model organisms and in nature that hsp genes, and hsp70 in particular, play an important role in adaptation to extreme or rapidly changing environmental conditions. During adaptive evolution, the hsp70 gene system in various eukaryotic organisms has undergone characteristic changes both at the level of structure, organization, and expression pattern of these genes in the genome and at the level of the structure of the Hsp70 family proteins themselves. Recent studies on the role of endogenous Hsp70 as well as recombinant Hsp70 in various diseases have demonstrated protective, anti-inflammatory functions of this protein to maintain proteostasis in the cells in various pathologies, including neurodegeneration and aging. The dual and sometimes antagonistic role of Hsp70 in cancer cells, fibrosis, and in infection with various viruses, including SARS, is discussed. The data accumulated in recent years represent a basis for the use of recHSP70 or its inducers in the therapy of many diseases.
HS, heat shock; Hsp, heat shock proteins; eHsp70, extracellular Hsp70; recHsp70,
recombinant Hsp70; HSF1, Heat Shock Factor; HSE, heat shock elements; RNAPII, RNA
polymerase II; D. melanogaster, Drosophila melanogaster; TLR2/4,
Toll-Like Receptor2/4; SR, scavenger receptors; EV, extracellular vesicles; BPD,
bronchopulmonary Dysplasia; AD, Alzheimer disease; ALS, amyotrophic lateral
sclerosis; NO, nitric oxide; HOP, Hsp70-Hsp90 Organizing Protein; ROS, reactive oxygen species; LPS, lipopolysaccharide; NF-
Conceptualization and supervision—MBE; Writing—MBE, OGZ; Editing—DGG, LNC, OGZ, SBO; Formal analysis—OGZ, LNC, DGG; Visualisation—DGG. All authors contributed to editorial changes in the manuscript. All authors read and approved the final manuscript.
Not applicable.
Not applicable.
The work was funded by Russian Science Foundation Grants 17-74-30030 (MBE) and 19-14-00167 (DGG).
The authors declare no conflict of interest.
Publisher’s Note: IMR Press stays neutral with regard to jurisdictional claims in published maps and institutional affiliations.