- Academic Editor
Background: Ganoderma lucidum spore powder (GLSP) has abundant
pharmacological activities. However, the difference in the hepatoprotective
function of sporoderm-broken and sporoderm-unbroken Ganoderma spore powder has
not been studied. This study is the first to investigate the effects of both
sporoderm-damaged and sporoderm-intact GLSP on the improvement of acute alcoholic
liver injury in mice and gut microbiota of mice. Methods: Serum
aspartate aminotransferase (AST) and alanine aminotransferase (ALT) levels and
interleukin 1
The liver is an important organ of human metabolism and the largest detoxification organ [1]. A number of studies have shown that after alcohol consumption, alcohol enters the blood after being absorbed through the gastrointestinal tract, and metabolic reactions occur in the liver. Long-term irrational eating and drinking habits can cause liver damage and lead to a range of liver diseases, such as alcoholic liver disease (ALD) [2]. ALD manifests as fatty liver in the early stage, which can then develop into alcoholic hepatitis, liver fibrosis, cirrhosis, and even liver failure [3]. ALD is the main cause of morbidity and mortality in industrialized countries and developing countries and is an advanced disease that is difficult to cure [4]. Therefore, it is important to study the mechanism of ALD and its treatment.
Various microbes reside in the intestinal tract of animals, and the number of microbes is very large. These microbes are very closely related to the physiological activities, metabolism, and immunity of the host. Under normal conditions, the gut microbiota and the host coexist harmoniously, and gut microbiota play important roles in maintaining a normal immune system, preventing pathogenic bacteria from colonizing the body, and the digestion and absorption of nutrients. When the gut microbiota composition changes, symbiotic disorders can occur, causing various diseases [5, 6, 7, 8, 9]. A large number of experiments have shown that the occurrence and development of liver diseases are closely related to changes in gut microbiota. Long-term alcohol abuse can change the structure and distribution of gut microbiota in the body, causing mucosal damage and bacterial translocation, reducing the number of beneficial bacteria, increasing the number of harmful bacteria, and aggravating liver cell damage [10, 11, 12]. Studies have shown that by regulating the diversity of the gut microbiota, the composition of the gut microbiota can be optimized, an increase in beneficial bacteria can be promoted, and the growth of pathogenic bacteria can be inhibited, thereby playing a protective role against alcoholic liver injury [13].
A large number of studies have shown that the active substances in mushrooms, such as Hericium erinaceus and Ganoderma lucidum, can be used as prebiotics to regulate gut microbes, thereby improving the health of the body [14]. Ganoderma lucidum spore powder (GLSP) is a type of spore ejected from the fruiting body of G. lucidum at the maturation stage [15, 16], has all the genetic material and health care functions of G. lucidum [17, 18], and is an extremely important medicinal and edible fungus. Modern pharmacological and clinical studies have shown that GLSP is rich in G. lucidum polysaccharides, triterpenes, proteins, and amino acids and is often used in the treatment of diseases, such as cancer, tumors, chronic liver diseases, insomnia, and low immunity [19, 20, 21, 22, 23, 24]. GLSP is an excellent regulator of microbes and can regulate the gut microbiota and improve the immunity of the body [25]. A large number of studies have shown that GLSP can reduce cell damage, promote liver cell regeneration, and play a protective role in the liver [26]. G. lucidum spores can be divided into sporoderm-broken and sporoderm-unbroken spores [27]. Studies have shown that the extraction rate of bioactive components and the biological effects of G. lucidum spores are improved [28]. To evaluate the hepatoprotective effect and ability to regulate gut microbiota of both sporoderm-broken and sporoderm-unbroken GLSP and to identify differences between sporoderm-broken and sporoderm-unbroken GLSP, this study established a model of acute liver injury in mice caused by 50% ethanol and analyzed the liver function, anti-inflammatory indexes, changes in gut microbiota, and pathological sections from mice treated with diammonium glycyrrhizinate (DG, positive control drug), sporoderm-broken GLSP and sporoderm-unbroken GLSP to provide a theoretical basis for the clinical application of GLSP.
Both sporoderm-broken (catalog number: 20181018) and sporoderm-unbroken (catalog number: 20181102) GLSP were purchased from Jilin Beizhi Biotechnology Co., Ltd. (Jilin, Jilin, China).
A total of 50 SPF grade C57BL/6 male mice, weighing 20
Sodium carboxymethyl cellulose (CMC) (catalog number: 9004-32-4), sodium
chloride injection (catalog number: 7647-14-5), and enteric-coated DG capsules
(catalog number: 79165-06-3) were purchased from Chia Tai‑Tianqing Pharmaceutical
Co., Ltd. (Nanjing, Jiangsu, China). Aspartate aminotransferase (AST), alanine
aminotransferase (ALT) (catalog number: 9000-97-9), interleukin-18 (IL-18)
(catalog number: 9000-86-6), leukocyte interleukin 1
An appropriate amount of dry sporoderm-broken and sporoderm-unbroken GLSP was added to 0.5% CMC solution and dissolved by ultrasonication for 20 min (KQ-500DA, Kunshan Ultrasound Instrument Co., Ltd., Kunshan, Jiangsu, China) to prepare a suspension with concentration of 6 mg/mL, which was stored at 4 °C for later use.
Fifty mice were randomly divided into 5 groups, with 10 mice in each group: the control group (CG), the 50% ethanol model group (MG), the positive control group treated with DG (DGG), the sporoderm-broken GLSP group (BG), and the sporoderm-unbroken GLSP group (UG). After 5 days of adaptive feeding, the CG and MG were given an equal volume of 0.5% CMC solution for 10 days; the DGG, BG, and UG were administered drug at 0.2 mL/10 g according to mouse body weight for 10 days; and mouse body weight was recorded daily. On the 9th day, at 2 h after drug administration, except for the CG, the mice in the other 4 groups were intragastrically administered 50% ethanol at 10 mL/kg twice at an interval of 12 h. The CG was given an equal dose of water. Twelve hours later, the mice were subjected to orbital blood collection and sacrificed by cervical dislocation. Liver and stomach tissues were dissected, washed, and stored in a –80 °C freezer for further analysis. After sacrifice, feces in the bowels of mice were collected into a sterile centrifuge tube, which was immediately placed in liquid nitrogen for quick freezing for the detection of gut microbiota. During the study, diet and water were not restricted, and the bedding was changed daily. The detailed experimental protocol and drug administration are shown in Fig. 1.
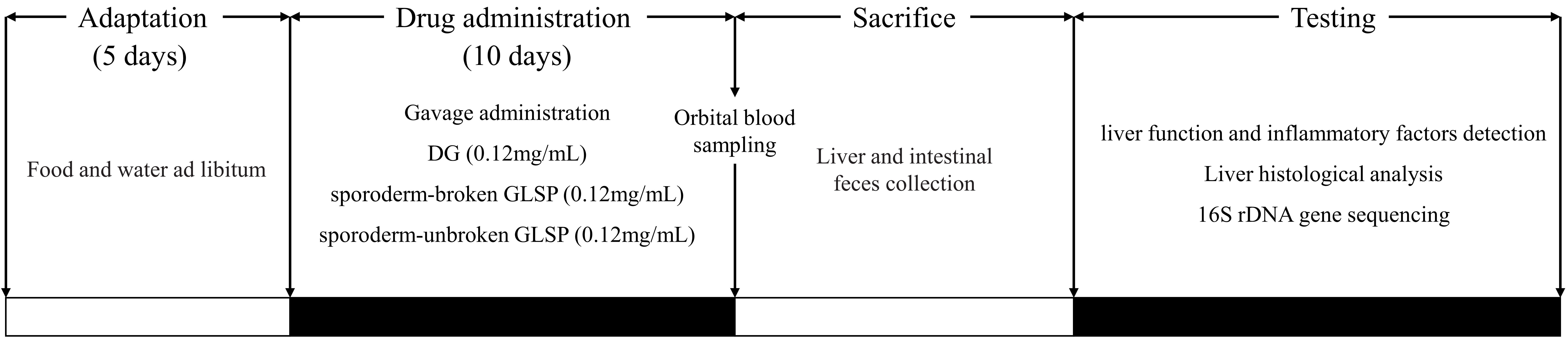
The experimental protocol and drug administration.
Whole blood was centrifuged at 5000 r/min for 10 min, and the serum was
collected to detect the levels of AST and ALT 10% liver homogenate was prepared
with 0.1 g of liver tissue and 0.9 mL of saline and centrifuged (TGL-16M, Hunan
Kaida Scientific Instruments Co., Ltd., Changsha, Hunan, China), and the
supernatant was taken for determination of IL-1
Liver tissues from the same part of the liver in each group were fixed with 10%
formaldehyde solution. The liver tissues were dehydrated, cleared, embedded in
paraffin, sectioned, and stained with hematoxylin-eosin (HE), and
histopathological changes in the liver were observed at 200
Fecal samples from 8 mice in each group were randomly selected and sent to
Shanghai Genesky Bio-Tech Co., Ltd. (Shanghai, China) for polymerase chain
reaction (PCR) amplicon sequencing. The integrity and quality of genomic DNA were
examined using agarose gel electrophoresis (DYCP-31DN, Beijing Liuyi Biotechnology Co., Ltd., Beijing, China) and a Nanodrop 2000 spectrophotometer (NanoDrop 2000, Thermo Fisher Scientific, Waltham, MA, USA).
Qualified samples with clearly visible electrophoretic bands and no significant
degradation were selected for high-fidelity PCR amplification. Sequencing was
performed using the MiSeq platform, followed by bioinformatics analysis.
TrimGalore, FLASH2, mothur, and usearch software were used to remove unusable
sequences, and the resulting paired sequences were spliced to identify and remove
primers. Finally, optimized sequences with high quality and reliability were
obtained. Sample Shannon curves, rank abundance distribution curves and species
accumulation curves were plotted to determine species abundance and species
evenness. Next,
Experimental results are expressed as the mean
AST and ALT levels in mouse serum are shown in Fig. 2. Compared with levels in
the CG, the serum AST and ALT levels in the mice in the MG were significantly
increased (p
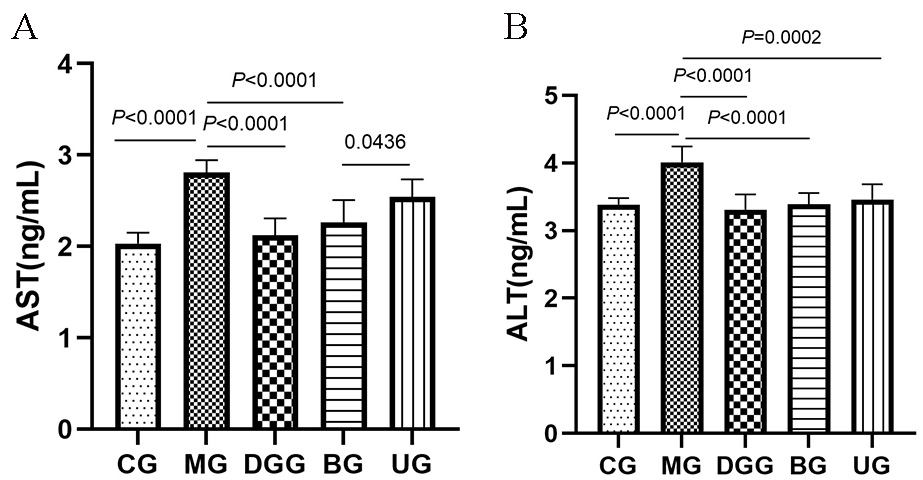
GLSP treatment affected the serum levels of (A) AST and (B) ALT in mice.
Fig. 3 shows that IL-1
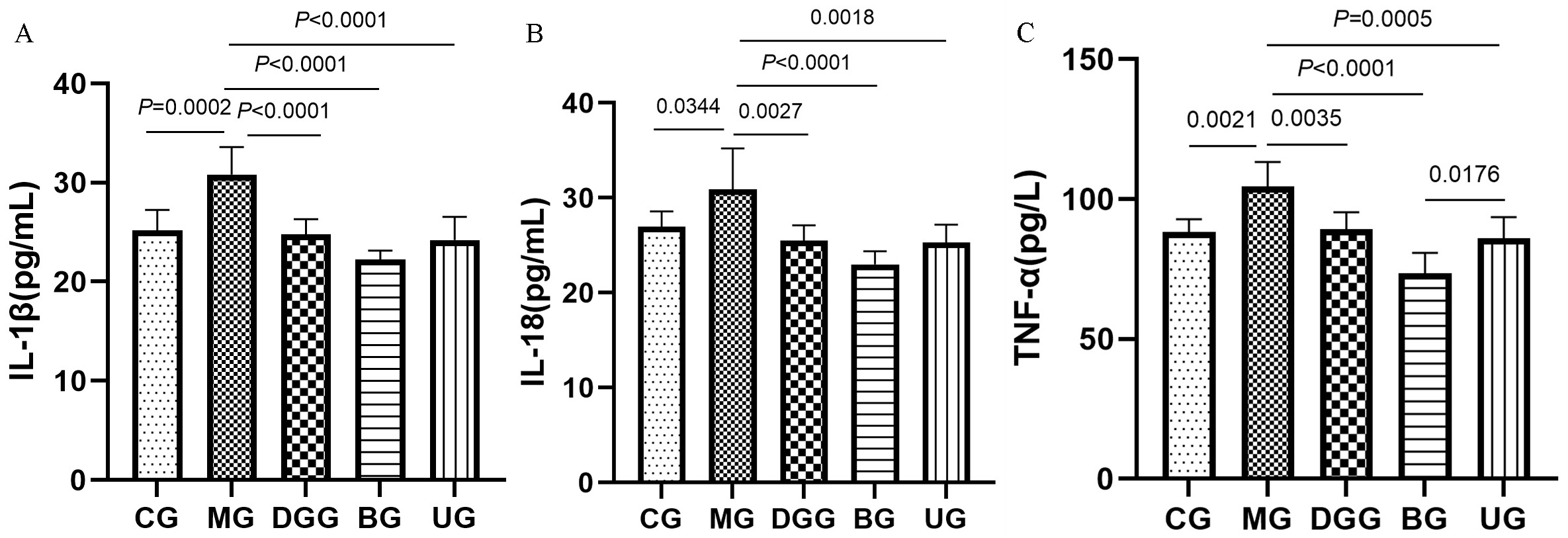
FLPs treatment regulated the levels of the (A) IL-1
HE-stained sections of liver tissues were observed under an optical microscope (Fig. 4), each group has two parallel liver histopathology sections. As shown in the figure, in the CG, the morphology of the liver cells was normal, the structure of the liver lobules was clear, the hepatic cords were neatly arranged, the liver sinusoids were not significantly dilated or squeezed, no swelling or inflammation was evident, and many hepatocytes with loose cytoplasm were observed, which may have been caused by glycogen-induced lysis. In the MG, edema to balloon-like degeneration, cytoplasmic vacuolization, and more common central vein and portal vein congestion and expansion were noted, and large areas of bleeding (yellow arrow) and white blood cells (blue arrow) were observed. In the GLSP-treated groups, no significant pathological changes were observed in liver cells, and hepatocytes were morphologically intact and well arranged, with more hepatocytes having loose cytoplasm and tending to exhibit a normal structure.
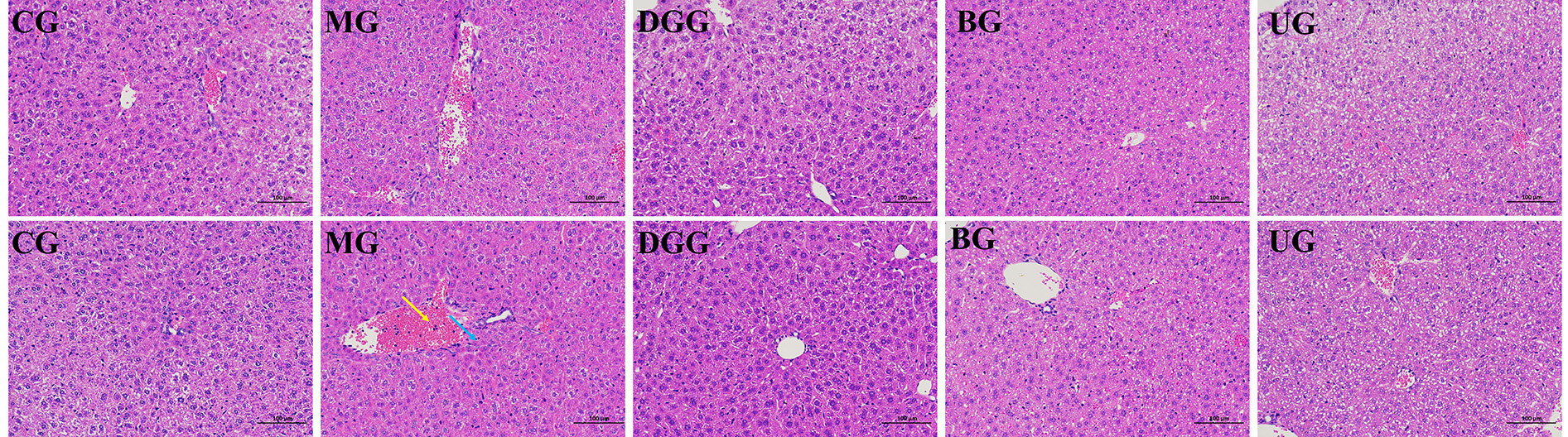
Pathological sections of liver tissues (H&E staining
Forty samples were sequenced to provide 15,501,589 raw sequences. The average sequence length was in the range of 400–500 bp, which matched with the sequence length of the 16S rDNA V3–V4 region. Therefore, the subsequent analysis was expected to provide reliable results. The Rank-abundance curve (Fig. 5A) can explain the species richness and community uniformity. In this study, all groups showed a large and gently decreasing range on the horizontal axis, indicating a high species richness and uniform species distribution of intestinal flora in mice in each treatment group. The Shannon curve (Fig. 5B) reflects the microbial community diversity, and its value is positively correlated with the community diversity. In this study, the curves of all groups tended to be flat, indicating that the amount of data met the sequencing requirements, and thus, the data comprehensively reflected the microbial diversity of the samples. Species accumulation curves (Fig. 5C) are used to depict the increase of species with increasing sampling size, and is widely used to determine the adequacy of sampling size. It is widely used to determine the adequacy of the sample size. The curve flattens out with the increase in the number of samples measured, indicating that the species in this environment do not increase significantly with the increase in the sample size and that the sampling size is adequate.
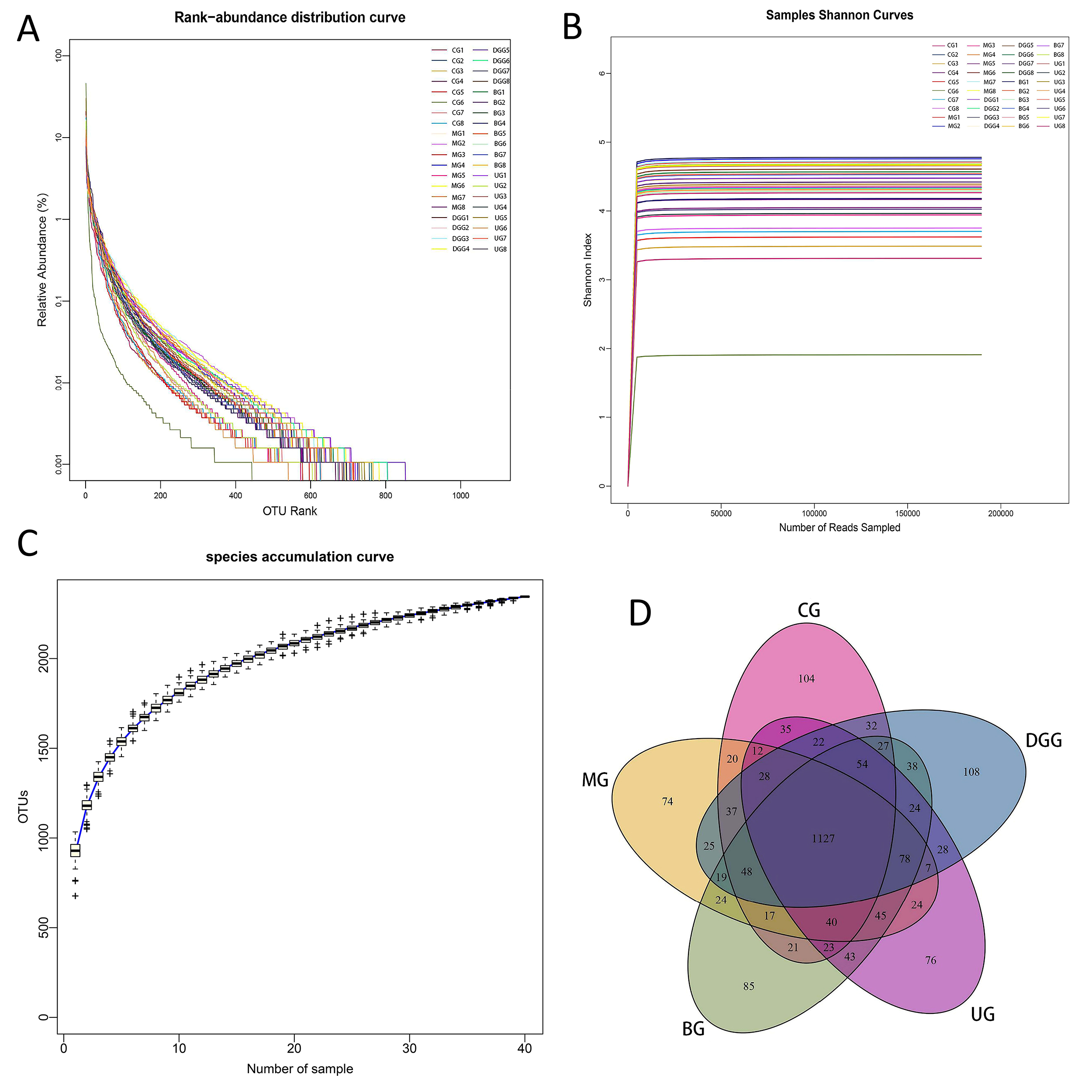
16S rDNA analysis of key operational taxonomic units (OTUs) of gut microbiota in mice. (A) Rank-abundance distribution curve. (B) Samples Shannon curves. (C) Quality analysis of sequencing result. (D) Venn diagram.
After clustering the gene sequences of mouse intestinal bacteria from each sample, which were obtained by high-throughput sequencing, they were grouped into different OTUs based on the similarity between sequences. The clustering and bioinformatics of OTUs were performed at a 97% similarity level, and a total of 2345 OTUs were obtained (Fig. 5D). The total number of OTUs shared by the 5 treatment groups was 1127. The number of OTUs specific to the MG was lower than that specific to the CG, while the number of OTUs specific to the BG was significantly higher than that specific to the MG and the UG. This finding indicates that different treatments can significantly affect the intestinal flora of mice.
The
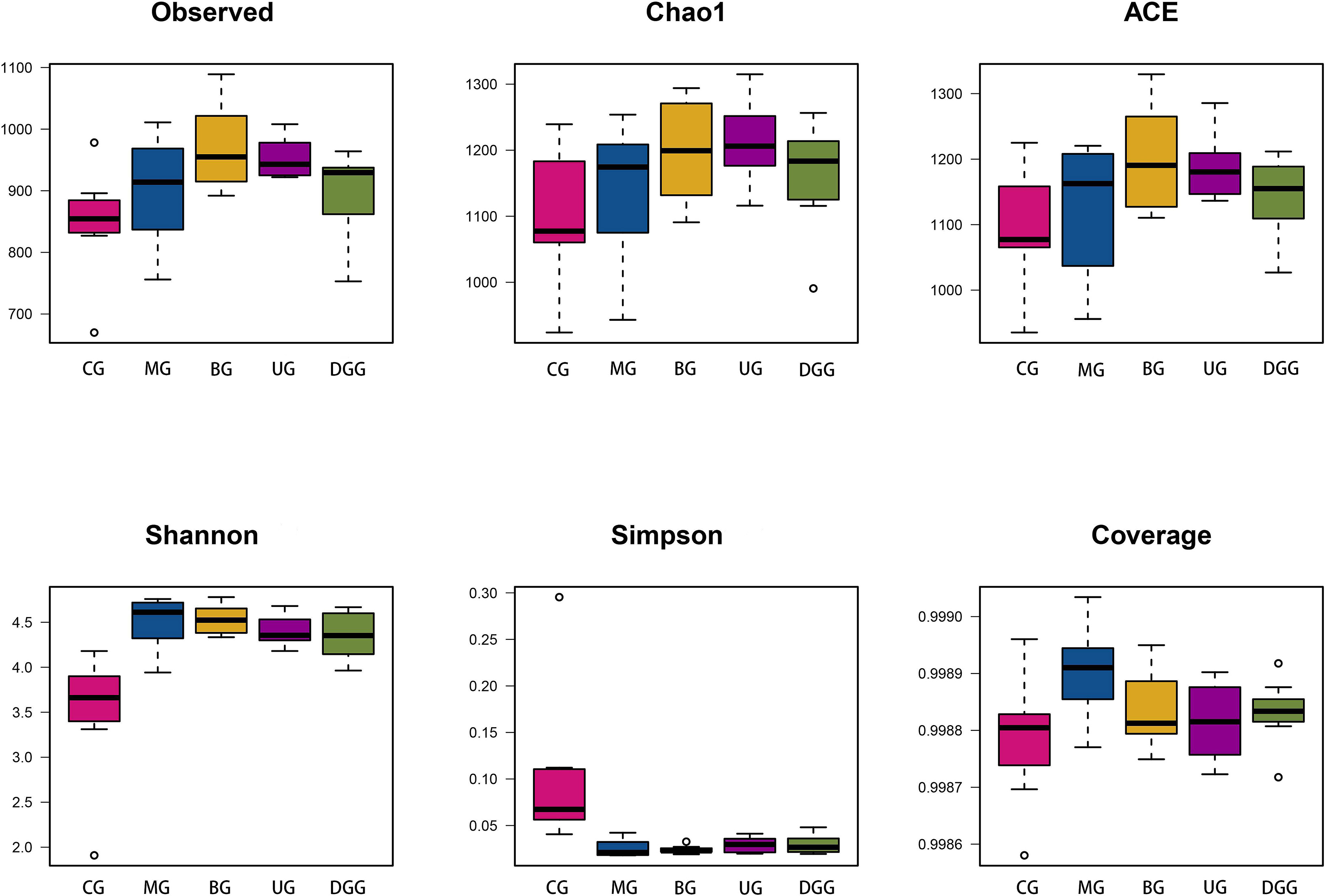
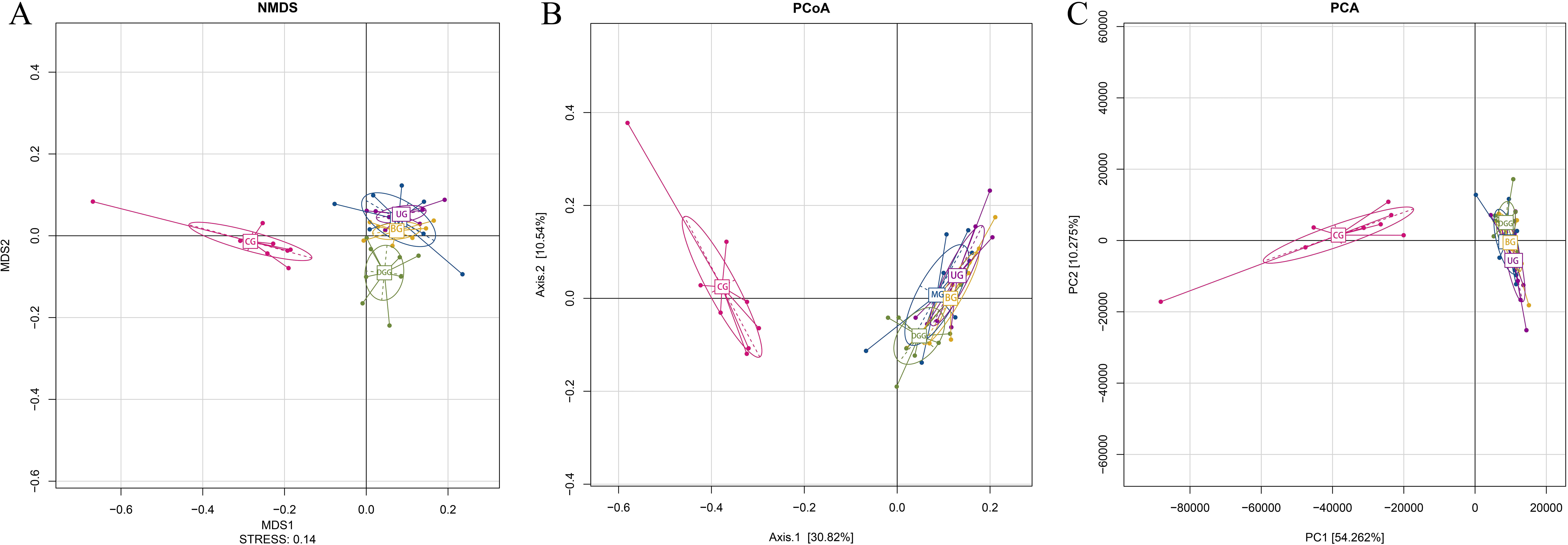
To visually observe the consistency of the species composition in each group and
differences in the species composition between groups, species with a relative
abundance
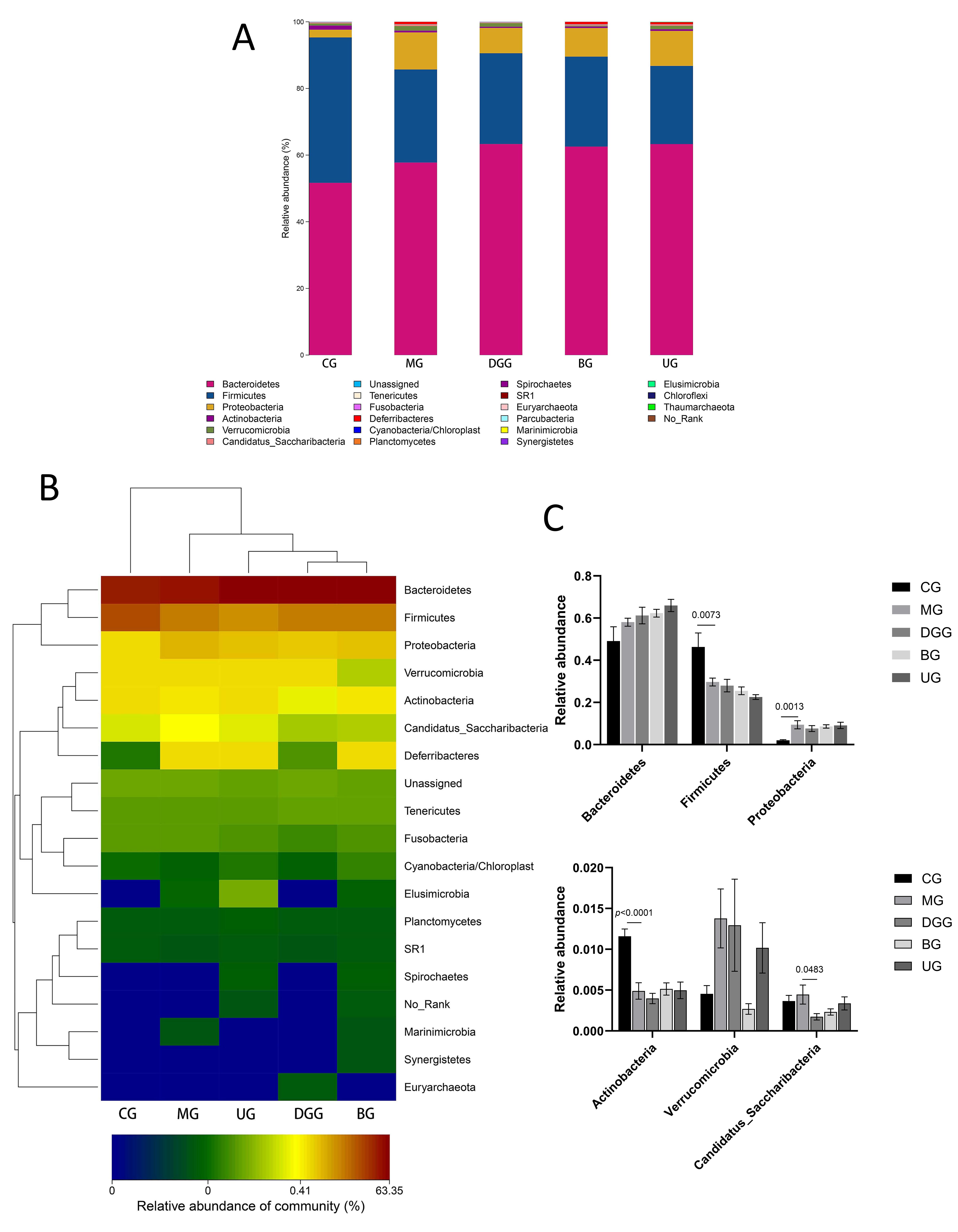
Effect of GLSP on phylum-level gut microbiota in mice. (A) phylum-level histogram. (B) Cluster heatmap of species abundance. (C) Quantitative statistics of bacteria with phylum-level differences.
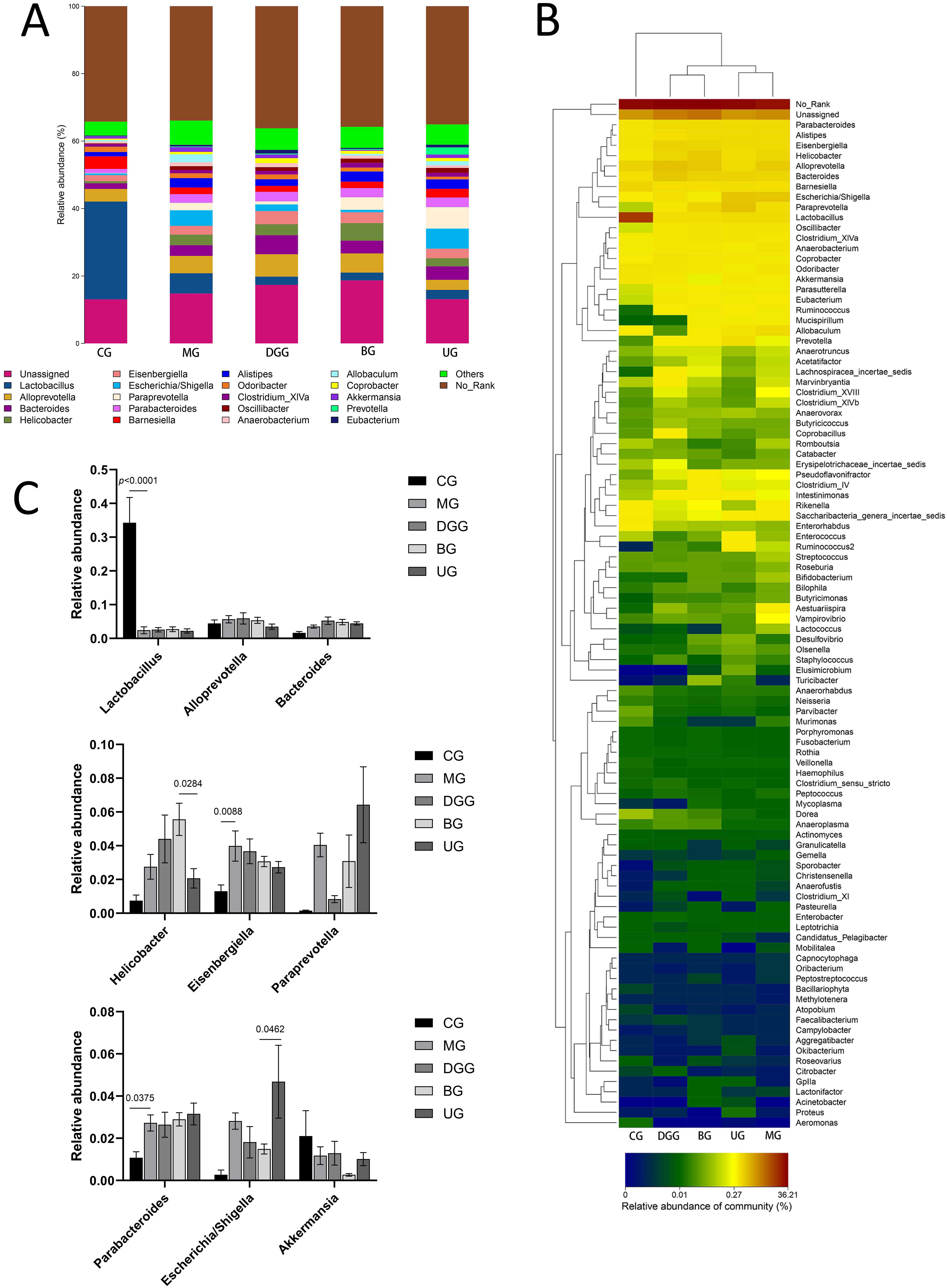
Effect of GLSP on the genus-level gut microbiota in mice. (A) Genus-level histogram. (B) Cluster heatmap of species abundance. (C) Quantitative statistics of bacteria with genus-level differences.
Fig. 8A,C show that at the phylum level, the dominant phyla of the mouse gut
microbiota were Bacteroidetes, Firmicutes,
Proteobacteria, Actinobacteria, and Verrucomicrobia,
with Bacteroidetes and Firmicutes representing more than 90%
of the microbiota. Compared with levels in the CG, the relative abundance levels
of Firmicutes (p = 0.0073) and Actinobacteria
(p
Fig. 9A,C show that the relative abundance of Lactobacillus was
significantly reduced compared with that in the CG (p
In addition, LEfSe analysis was performed on these five groups of mice for the
simultaneous identification of specific taxa across phylum, class, order, family
and genus levels. The histogram of LDA effect values for biomarker species is
shown in Supplementary Fig. 1. It was found that there were 65
significantly different species in CG (LDA
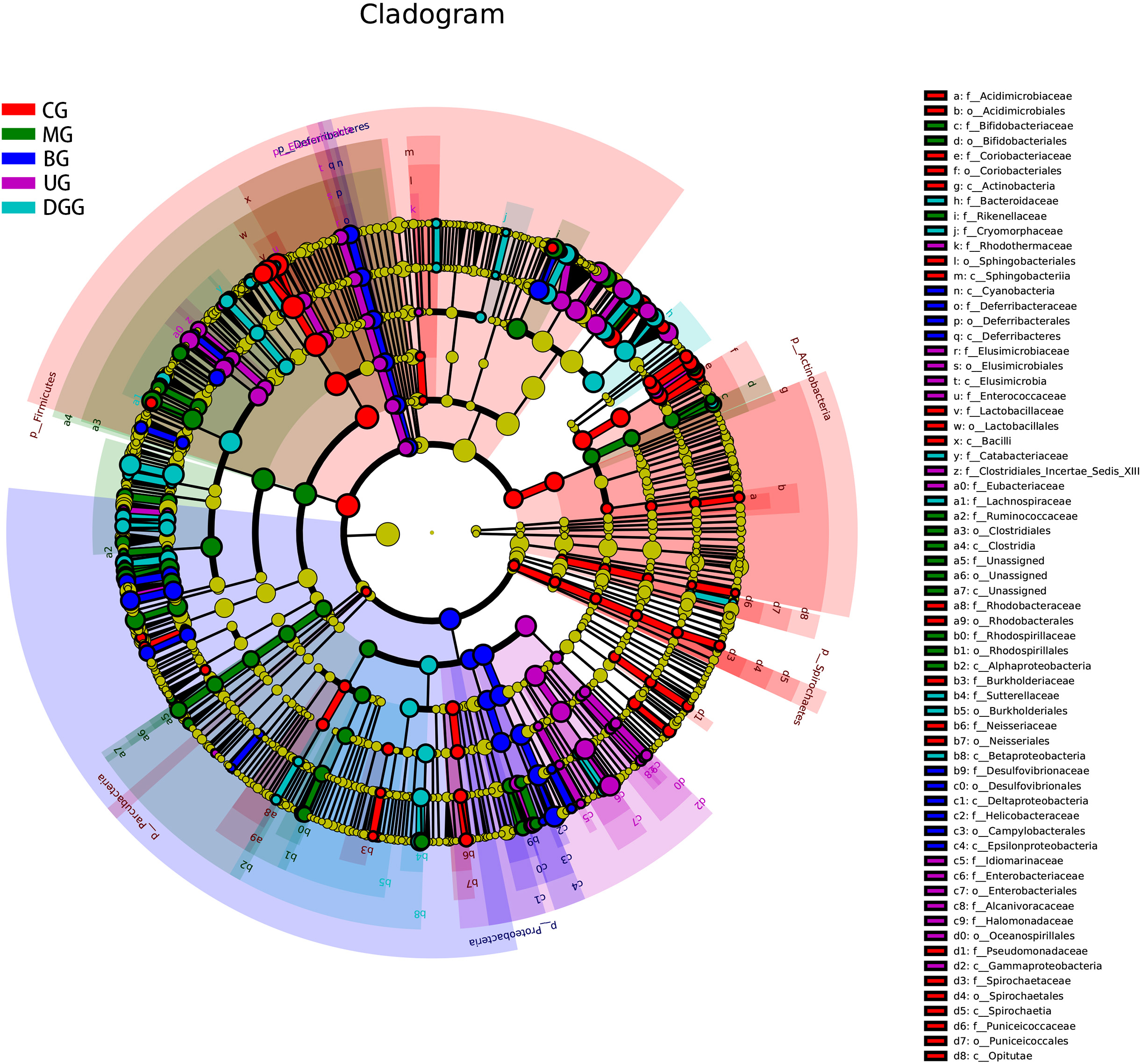
Linear discriminant analysis effect size cladogram for the comparisons of the five study groups.
Fig. 11 shows the analysis of differences in clusters of orthologous groups (COG) composition, listing the functional categories in which COG composition differed significantly between groups and the proportion in each group, with the proportion of differences and confidence intervals and p-values given on the right. Nucleotide transport and metabolism (p = 0.01), translation, ribosomal structure and biogenesis (p = 0.014), and lipid transport and metabolism (p = 0.015) were significantly downregulated in the MG mice intestinal flora compared to CG. Significant upregulation of coenzyme transport and metabolism (p = 0.01), translation, ribosome structure and biogenesis (p = 0.014), cell wall/membrane/envelope biogenesis (p = 0.02), and post-translational modifications, protein turnover and chaperones (p = 0.023) in BG mice intestinal flora compared to MG. The functions of lipid transport and metabolism (p = 0.017), coenzyme transport and metabolism (p = 0.024), and cell wall/membrane/envelope biogenesis (p = 0.026) were significantly upregulated in the intestinal flora of UG mice.
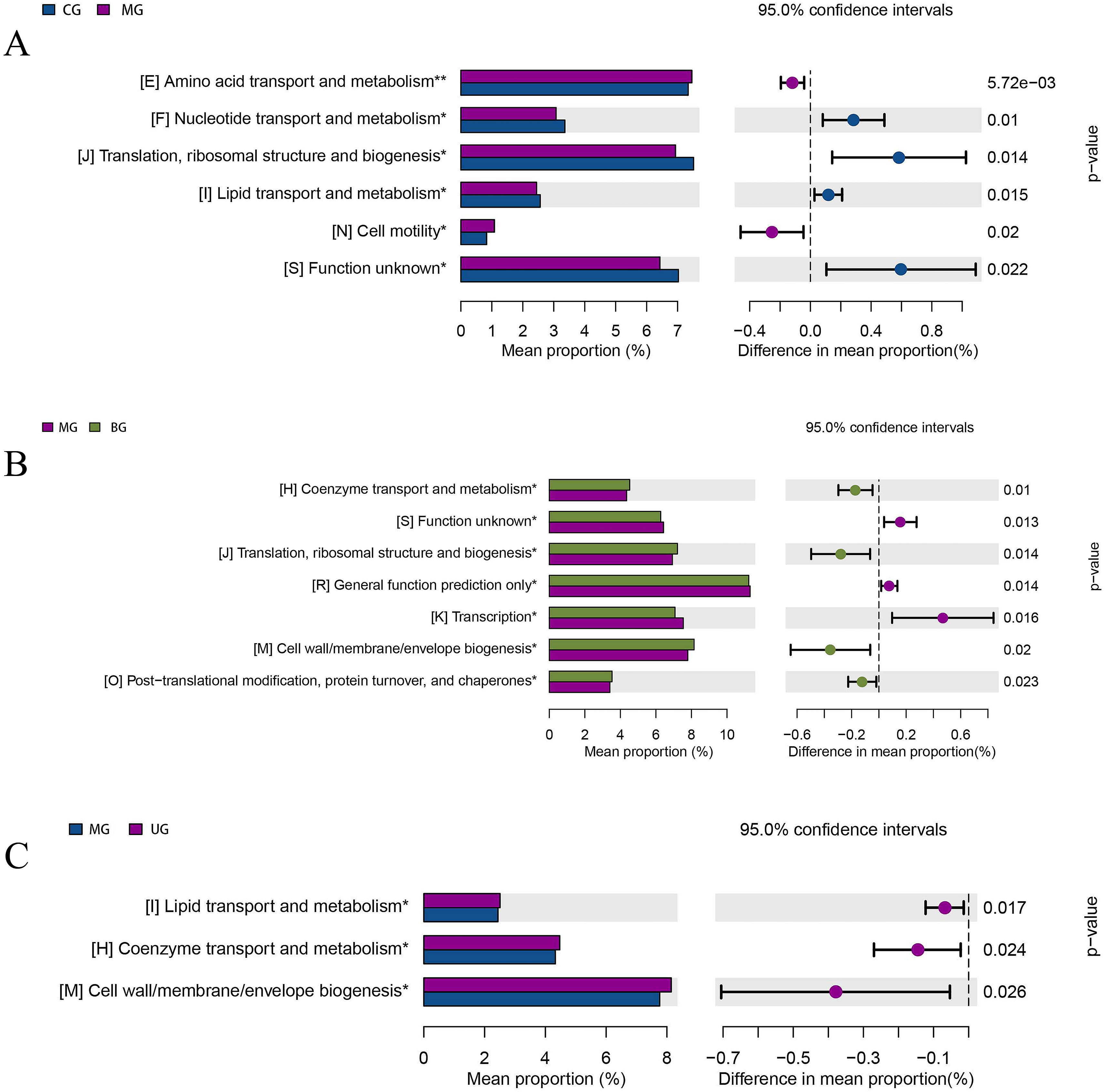
COG function enrichment analysis of gut microbiota of mice. (A) CG versus MG. (B) MG versus BG. (C) MG versus UG.
The kyoto encyclopedia of genes and genomes (KEGG) database is a large knowledge base for systematic analysis of gene functions, linking genomic information and functional information. As shown in Fig. 12, the levels of 65 functions such as vibrio cholerae pathogenic cycle (p = 0.0005), bacterial motility proteins (p = 0.0007), and bacterial chemotaxis (p = 0.0011) were significantly downregulated in the intestinal flora of MG mice compared with CG; compared with MG, the levels of 25 functions such as biotin metabolism (p = 0.0041), restriction enzyme (p = 0.015), carbon fixation pathways in prokaryotes (p = 0.017) were significantly upregulated in BG mice intestinal flora, and the levels of 30 functions such as biotin metabolism (p = 0.0099), carbon fixation pathways in prokaryotes (p = 0.013) and peroxisome (p = 0.015) were significantly upregulated in UG mice.
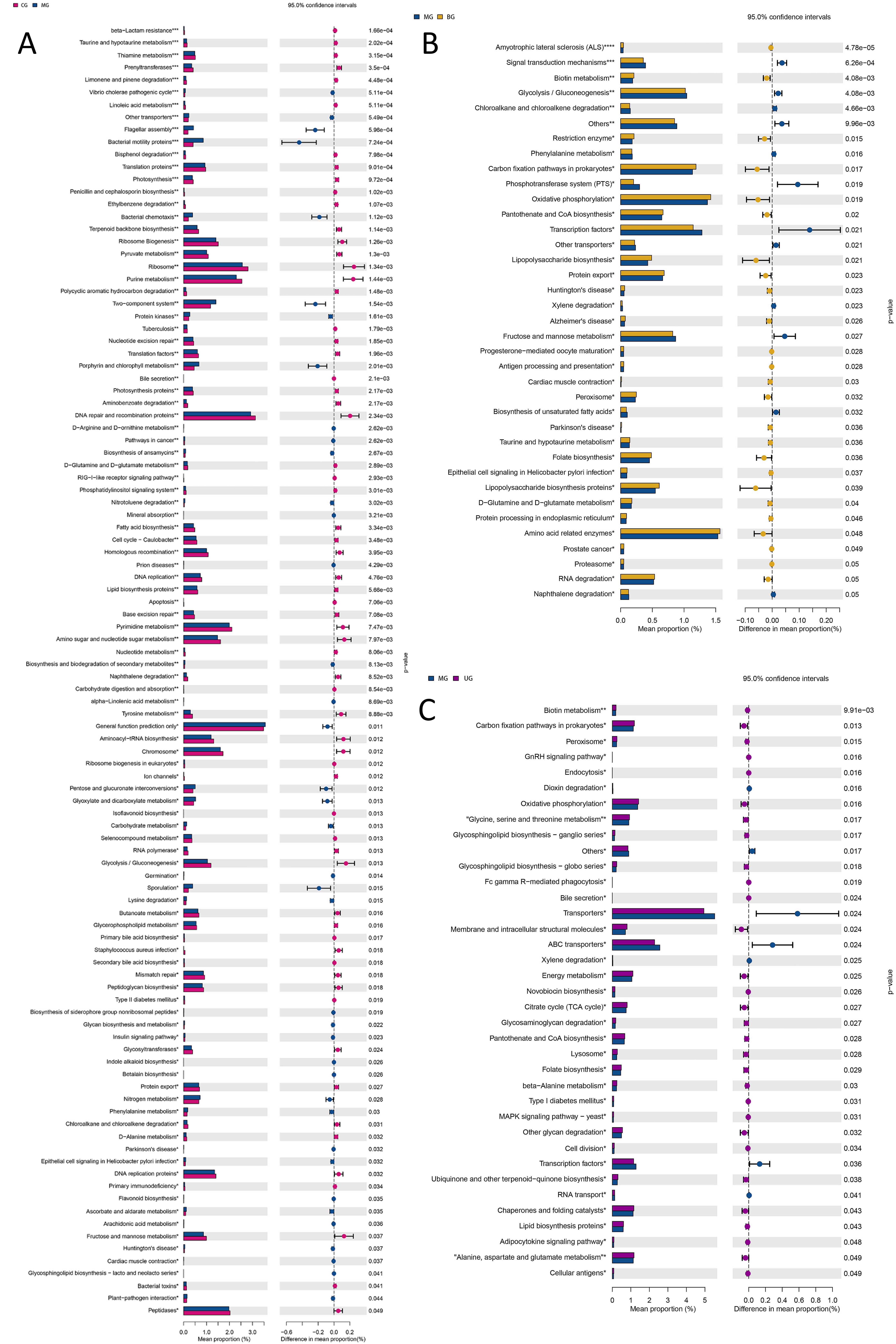
KEGG function enrichment analysis of gut microbiota of mice. (A) CG versus MG. (B) MG versus BG. (C) MG versus UG.
In an experimental model of acute alcoholic liver injury, this study demonstrated that GLSP may play a beneficial role in protecting the liver by regulating the gut microbial community structure.
In the normal environment of the body, AST and ALT are present in small amounts in liver cells. When cells are damaged, cell membrane permeability is enhanced, a large amount of AST and ALT are released into the blood, and their concentrations in serum are increased. Therefore, AST and ALT are often used as important indicators for the diagnosis of viral hepatitis and toxic hepatitis [29, 30, 31]. In this study, the BG showed significantly reduced serum AST and ALT levels caused by alcohol, but the UG showed a significant inhibitory effect only on the increased ALT level caused by liver injury and no significant effect on AST, indicating that in the BG and UG, the changes in AST and ALT levels caused by alcohol-induced liver injury were regulated, with a better effect in the BG.
Long-term intake of alcohol can lead to a sudden increase in microbial products,
such as endotoxin in the blood, and the phagocytic cells located on the inner
surface of liver sinusoids, such as Kupffer cells, bind to endotoxin and are
activated; thus, a large number of cytokines and inflammatory mediators, such as
IL-1
To investigate the effect of GLSP treatment on the intestinal flora of mice with acute alcoholic liver injury, 16S rDNA gene sequencing was performed on the colonic feces of mice in each treatment group. The diversity analysis of intestinal flora of mice revealed that sporoderm-broken GLSP could significantly improve the abundance and diversity of intestinal microorganisms in liver-injured mice. A number of studies have shown that long-term alcohol stress can change the intestinal permeability in the body, thereby increasing the number of Bacteroidetes and Verrucomicrobia in the cecum and reducing the growth of bacteria with anti-inflammatory activity, such as Firmicutes [38]. Bacteroidetes is the second most dominant phylum in the intestine, participates in the metabolism of carbohydrates and lipids in the intestine, and has a very important impact on the health of the host [39]. Firmicutes can participate in carbohydrate metabolism and maintain healthy gut microbiota [40]. The relative abundance ratio of Bacteroidetes to Firmicutes is an important indicator of changes in microbiota composition, and changes in this indicator may lead to the occurrence of complications, such as obesity, diabetes, inflammatory bowel disease, and liver disease [41, 42, 43, 44]. Studies have shown that G. lucidum polysaccharides can reverse Bacteroidetes/Firmicutes levels, increase anti-inflammatory bacteria and short-chain fatty acid-producing bacteria, reduce some disease-related bacteria, and remodel the gut microbiota [45]. In this study, compared with levels in the CG, the Bacteroidetes/Firmicutes levels in the MG were significantly increased, indicating that alcohol caused an imbalance in the gut microbiota in mice and that the model was established successfully. Compared with the MG, the Bacteroidetes/Firmicutes levels in the DGG, BG, and UG did not show significant changes, indicating that GLSP and the positive control drug did not alleviate alcoholic liver injury by reversing the Bacteroidetes/Firmicutes levels. Compared with that in the CG, the level of Lactobacillus in the MG was significantly reduced, which is consistent with the results of Hartmann et al. [46], indicating that the occurrence of ALD may be related to a decrease in the relative abundance of Lactobacillus. Studies have shown that the Proteobacteria level in the intestines of most diseased hosts is higher than that in healthy hosts, and a large amount of proinflammatory factors are released, which increases intestinal permeability, destroys the redox balance in the intestine, causes endotoxin leakage into the plasma, and eventually causes liver steatosis, inflammation, and apoptosis [47, 48, 49, 50]. Escherichia_Shigella can adversely affect liver lipid metabolism [51]. Zhao et al. [52] found that increases in the relative abundance levels of Proteobacteria and Escherichia_Shigella in the intestine of rats can cause liver damage. In the present study, compared with levels in the CG, the relative abundance levels of Proteobacteria and Escherichia_Shigella in the MG were increased. Compared with levels in the MG, the relative abundance levels of Proteobacteria and Escherichia_Shigella in the DGG and BG showed a decreasing trend, the inhibitory effect on Escherichia_Shigella in the BG was better than that in the DGG, and the level of Escherichia_Shigella in the BG was close to the normal level in the CG, indicating that the sporoderm-broken GLSP alleviated the alcohol-induced increases in Proteobacteria and Escherichia_Shigella levels in the intestine, thereby achieving a liver-protecting effect. Evidence indicates that the relative abundance of Candidatus_Saccharibacteria in the intestines of alcohol-treated mice may increase [53, 54, 55]. Candidatus_Saccharibacteria invades the human skin, mucous membranes, and internal organs, resulting in a variety of acute and chronic diseases, such as interdigital erosion, rash, thrush, intracardiac encephalitis, and meningitis [56]. In the present study, compared with the CG, the Candidatus_Saccharibacteria level in the MG was increased but not significantly, and after BG treatment, the relative abundance of Candidatus_Saccharibacteria decreased to the normal level, which may be another means to protect the liver. Analysis of the phylum- and genus-level heatmaps of the gut microbiota of mice in each group showed that sporoderm-broken GLSP increased abundance levels in the mice in the liver injury model, and the cluster analysis of the microbiota showed that the regulatory effect of sporoderm-broken GLSP on the genus-level gut microbiota was most similar to that of the positive control drug. In addition, the functions of the identified microbiota were predicted, and the levels of functions such as translation, ribosome structure and biogenesis, and lipid transport and metabolism were found to be down-regulated in the intestinal flora of mice with acute liver injury, and GLSP treatment upregulated the levels of these functions; therefore, we speculate that these functions may also play an important role in the development of acute alcohol liver injury. In summary, alcohol consumption can significantly change the gut microbial abundance and diversity, but sporoderm-broken GLSP can partially regulate these changes and effectively ameliorate alcoholic liver injury.
This study investigated the ability of both sporoderm-broken and
sporoderm-unbroken GLSP to mitigate alcoholic liver injury in mice. The results
showed that GLSP reduced the increased AST and ALT levels in the blood caused by
liver injury; decreased the release of inflammatory factors, including
IL-1
The datasets used and analyzed during the current study are available from the corresponding author on reasonable request.
HW and SW—designed the research study. FW—performed the research. YL—conducted the study and drafted the manuscript. XW, XL and YL—analyzed the data. CC—provided administrative support. All authors contributed to editorial changes in the manuscript. All authors read and approved the final manuscript.
This experiment was approved by the ethics committee of Changchun University, in accordance with the relevant provisions of the national ethics of animal use in research (approval number: 20190126).
The authors thank the Institute of Ginseng Science of Changchun University of Traditional Chinese Medicine for its support of the study.
This study was supported by Jilin Province Science and Technology Development Plan Project (No. 20210401121YY), the Training Plan for Young Scientists of “Xinglin Scholar Project” of Changchun University of traditional Chinese Medicine (QNKXJ2-2021ZR03), and Jilin Province local herbal standardization project (JLPZGF-2018-005).
The authors declare no conflict of interest.
Publisher’s Note: IMR Press stays neutral with regard to jurisdictional claims in published maps and institutional affiliations.