- Academic Editor
Background: The medial prefrontal cortex (mPFC) is synaptically coupled to locus ceruleus (LC) located in the pontine tegmentum. The LC supplies norepinephrine (NE) to most of the central nervous system (CNS) via an elaborate efferent network. NE release in the cortex and various limbic structures regulates arousal, memory processes, adaptive behavior and cognitive control. Methods: The study investigated the role of the mPFC-LC circuit in the cognitive behavior of mice. The mPFC efferents were inhibited optogenetically at the level of dorso-rostral pons by virally delivered ArchT opsin. The mice were implanted bilaterally with optic fibers transmitting yellow light and tested for anxiety-like behavior on Elevated O-maze (EOM), for long-term memory with Novel Object Recognition test (NOR), for problem-solving ability with Puzzle test and for learning with Cued Fear Conditioning (FC). In addition, we used anterograde transsynaptic viral tracing to map a possible anatomical circuit allowing the mPFC to modulate the activity of LC neurons, which supply NE to the main limbic structures with a functional role in cognitive behavior. Results: The application of yellow light did not affect the anxiety-like behavior of the mice but impaired their ability to recognize a novel object and solve a problem. Optogenetic inhibition of mPFC to LC, in either acquisition or recall phase of FC similarly decreased freezing. The viral tracing identified the following tripartite circuits: mPFC-LC-dentate gyrus of the hippocampus (DG), mPFC-LC-amygdala (Amy), and mPFC-LC-mPFC. Conclusions: Our results reveal essential long-range regulatory circuits from the mPFC to LC and from LC to the limbic system that serves to optimize cognitive performance.
The medial prefrontal cortex (mPFC) projections can have excitatory and inhibitory effects on locus ceruleus (LC) neurons [1, 2], making direct synaptic contact not only with LC neurons but also with the neurons in the surrounding peri-LC dendritic area [3]. The presumptive functional role of the mPFC projections to LC is regulation of both the tonic and phasic noradrenergic activities, where the first is responsible for disengagement from a current task and the last for optimization of neuronal activity during a specific task [4]. The LC is the principal source of norepinephrine (NE) to the central nervous system (CNS) and is comprised of distinct populations of cells that are highly integrated with one another, and each LC sub-region receives region-biased input. The sub-regions of LC can independently activate and release NE in distinct brain regions [5, 6]. An example of this heterogeneous activity can be seen with activation of LC projections to the amygdala (Amy) during fear learning but LC projections to the mPFC during fear extinction [7].
Memories for aversive events are resilient and persist over time [8]. Cued fear
conditioning is a standard laboratory paradigm for studying the acquisition,
consolidation, recall, and extinction of fear memories. The test relies on the
association of an aversive unconditioned stimulus (US), such as mild electric
shock and a neutral conditioned stimulus (CS), such as tone. Over pairings, the
CS acquires aversive value and creates a memory that triggers freezing in rodents
during subsequent CS alone presentations, for review see [9]. The brain circuits
engaged in associative process are well established and include auditory and
nociceptive cortical and thalamic areas that project to the Amy, where the
presentation of the two sensory inputs causes long-term potentiation (LTP) [10]
and accelerates synaptogenesis [11, 12]. Both LTP and accelerated synaptogenesis
are enhanced by glucocorticoids and NE [13, 14]. Within the Amy, the role of NE
in memory consolidation is executed via the activation of
While the importance of NE from the LC for optimal performance of various cognitive tasks is well described, the exact inputs responsible for adjusting LC activity for specific cognitive tasks do not have the same level of clarity. The mPFC sends robust projections to the LC, and activation of these mPFC projections can serve as a strong modulatory input in the LC. Yet, little is known about the role of these projections in learning and memory. The present studies use a detailed circuit-based approach to determine the participation of the mPFC projections to the LC in various cognitive activities.
Adult outbred male mice, CD1 (cat. 022, Charles River, Wilmington, MA, USA), 8 to 10 weeks old and average weight of 30 grams were used in the study. The animals were housed in conventional cages in an AAALAC-accredited animal care facility and kept at 12:12 h light/dark cycle with food and water available ad libitum. All animal work complies with the guidelines set by the IACUC at Rosalind Franklin University of Medicine and Science, protocol number T23-10.
All behavior tests were done with mice implanted with optogenetic fibers attached to a light transmitter. The light transmitter was off in the control groups and switched on in the experimental groups, delivering yellow light. Behavioral tests were each separated by three to five days. The mice were randomly assigned to groups for different tests; therefore, each mouse had an equal chance to be in the control or experimental group.
Elevated O-maze (EOM) is a continuous circular track (approximately 10 cm wide
and 50 cm above the floor) with two open areas and two enclosed areas of the
track (each 50 cm
The mice were habituated to the testing arena (open field box for rats) in two
5-minute sessions. During testing, the optogenetic light emitters were attached
to the mice fiber optic cannula implants, and mice were placed in the testing
arena for five minutes with two objects similar in texture and size but not in
color. Objects were smaller than the mice. Objects were impermeable plastic
labware (e.g., test tube holders, caps, etc.) that were cleaned and sanitized
between each testing session. Mice were returned to their home cage for 3 hours
and then placed in the testing arena with one original and one novel object to
quantify recognition memory. The light transmitters were turned on in the
experimental group but remained off in the control animals. The session was
videorecorded and the amount of time investigating each object (the mouse nose is
less than 2 centimeters from the object) was automatically measured by Any-Maze
tracking software (Stoelting Inc., Wooddale, IL, USA). A discrimination index (DI)
expressed as a percent was used to estimate memory retention (DI =
(Time
The Puzzle Test evaluates goal-oriented behavior, relying on natural rodent avoidance of open, bright spaces [24, 25, 26]. We used a simplified protocol based on the Puzzle paradigm, combining a five-minute habituation phase followed by a problem-solving phase with a single obstacle [3]. The test was conducted in a modified mouse light-dark box where the dark and the light compartments were connected with a tunnel made of a plastic tube (15 cm long and 7 cm in diameter). The tube size was chosen to accommodate the headgear of the animals. The mice were left to explore the compartments for five minutes. Next, mice were briefly removed from the box and a barrier made of paper, which the mice could easily pull or push out of the tunnel, was used to block the entrance to the tunnel [26]. The mice were returned to the open, bright compartment, and the time to remove the barrier and enter the dark chamber was measured. The light transmitters of the experimental group were on during the problem-solving phase of the test.
The animals were placed in a commercial conditioning apparatus designed for mice (Any-Maze, Stoelting Inc., Wooddale, IL, USA). The apparatus is a Plexiglas container with metal bars making up the floor, a speaker in the wall, a camera mounted to the ceiling, and changeable walls in different patterns. Mice were habituated to the test box (Box A) in three separate sessions, each lasting 180 seconds. Test Box B was placed in a different room with different wall patterns, grid floor, and cleaning solution for the fear conditioning phase. After three minutes in Box B, the mice were exposed to an 80 dB, 30-second tone immediately followed by a single, two-second 1.5 mA electric shock. The animals remained in the box for another 2 minutes. Seven days later, the mice were tested for fear recall in Box A, where the conditioned stimulus (CS) with duration 30 seconds was presented three minutes after the mice entered the box. The mice were left in the box for an additional five minutes. Any-Maze tracking software (Stoelting Inc., Wooddale, IL, USA) quantified the animals’ freezing as a percent of the total time during the conditioning and testing sessions.
Two fear conditioning (FC) tests were performed using different groups of mice. In the first test, the yellow light was on during the acquisition phase (Box B) and off during the recall phase (Box A). In the second FC test, the light was off during the acquisition (Box B) and turned on in the recall phase (Box A). The control groups had mounted headgear, but the light transmitters were off for both phases of the experiment.
Mice were anesthetized with isoflurane (3% induction and 1–2% for
maintenance, catalog number 792632, Millipore-Sigma, Burlington, MA, USA). After
confirming anesthesia by tail pinch, the mice were placed in the stereotactic
frame (Stoelting Inc., Wood Dale, IL, USA) and the skull was exposed via a
midline incision. A 10 µL Hamilton syringe with a stainless-steel 32-gauge
needle was lowered into the region of interest through an opening drilled through
the skull according to the respective coordinates from bregma (LC:
anteroposterior (AP) – 5.4, lateral
For optogenetic silencing of the mPFC-LC pathway, we used an AAV viral construct
(pAAV
The same stereotaxic procedure was used to trace the tripartite mPFC – LC –
limbic regions circuit. Five mice of each group were injected with
AAV
Three weeks after the injection of AAV-ArchT, mice were implanted in the LC with a fiber optic cannula, bilateral, 250 µm diameter, 4.5 mm long, 6.2 mm distance center to center, emitting yellow LED light (590 nm wavelength; Elcom, San Diego, CA, USA). After recovering from surgery, the mice underwent three habituation sessions with headgear (dummy or functional receivers; Wireless receivers, TeleR-1-P, Teleopto, Amuza Inc., San Diego, CA, USA). ArchT is an inhibitory opsin-driven proton pump [28] that requires continuous illumination with low-intensity light [29]. Therefore, to prevent overheating and avoid tissue damage, we set up our pulse generator (STOmk-2, Amuza Inc., San Diego, CA, USA) to 10 ms light pulses with 5 Hz frequency and adjusted the light intensity to 15 mW at the cannula tip as measured by a light power meter, LPM-100 (Amuza Inc., San Diego, CA, USA). Mice with attached headgear were placed in the respective test equipment and the pulse generator was turned on. In the experimental groups, the light emission continued for the entire duration of the tests or 5 minutes. In control groups, the headgear was attached but the light transmitter switched off. The mice were perfused after the completion of the experiments to verify fiber optic cannula placements.
The mice were anesthetized by an injection of sodium pentobarbital (40 mg/kg
i.p., catalog number VED111, Penn Veterinary Supply, Inc., Lancaster, PA, USA).
After confirming mice no longer responded to tail pinch, mice were transcardially
perfused with 20 mL of phosphate-buffered saline (phosphate-buffered saline, PBS:
10 mM Na
Graph Prism 9 (GraphPad Dotmatics, Boston, MA, USA) software was used for all statistical analysis.
T-tests were applied to compare the behavior experiments with the
exception of FC experiments, where the freezing response during training and
testing phases was analyzed with a Two-way
analysis of variance (ANOVA) followed by post
hoc Tukey’s multiple comparison test with
The mPFC regulates visceral and autonomic responses via activation of robust
projections to the hypothalamus and brainstem centers, including the LC [30]. The
role of LC-NE in general arousal, attention, learning, memory, and stress is well
established. Here, we tested if inhibition of the mPFC projections to LC would
impact similar behaviors. The mice were injected bilaterally into the mPFC with
AAV-ArchT-GFP, an inhibitory proton pump, and were implanted with fiber-optic
cannula capable of delivering yellow light to the LC (Fig. 1, Ref. [31]). Mice were first
tested for anxiety-like behavior in the Elevated O-maze. There was no difference
between control (light off; 28.1
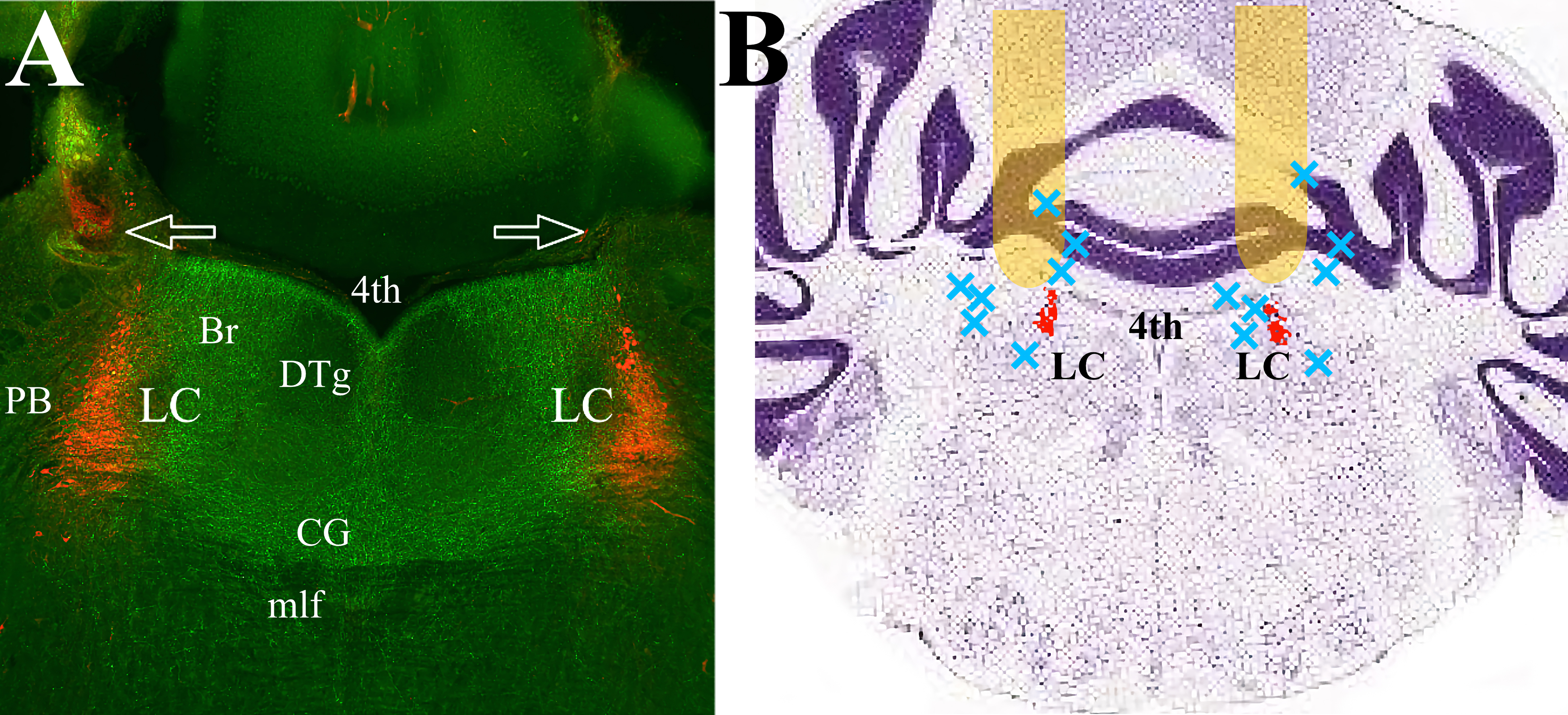
mPFC efferents cover the pontine tegmentum, including LC proper and peri-LC area. (A) The mPFC efferents expressing ArchT (green) traverse LC (red) and adjacent dendritic regions on both sides. There is minimal expression in the lateral and ventral parts of the pons. Arrows indicate fiberoptic tracks. (B) Schematic representation of the placement of the optic fibers. Behavioral data was collected from twenty-three mice with fiber optic cannula tracks positioned in the yellow areas. Seven animals with placements at approximately the blue X marks were excluded from analysis. LC proper is highlighted in red. The Nissl-stained coronal brain section is adapted from “Allen Brain Atlas”, (http://atlas.brain-map.org/atlas?atlas=1&plate=100960184). Abbreviations: 4th, Fourth ventricle; Br, Barrington nucleus; CG, Central Gray; DTg, Dorsal Tegmental nucleus; LC, Locus Ceruleus; mlf, medial longitudinal fasciculus; PB, parabrachial nucleus; mPFC, medial prefrontal cortex.
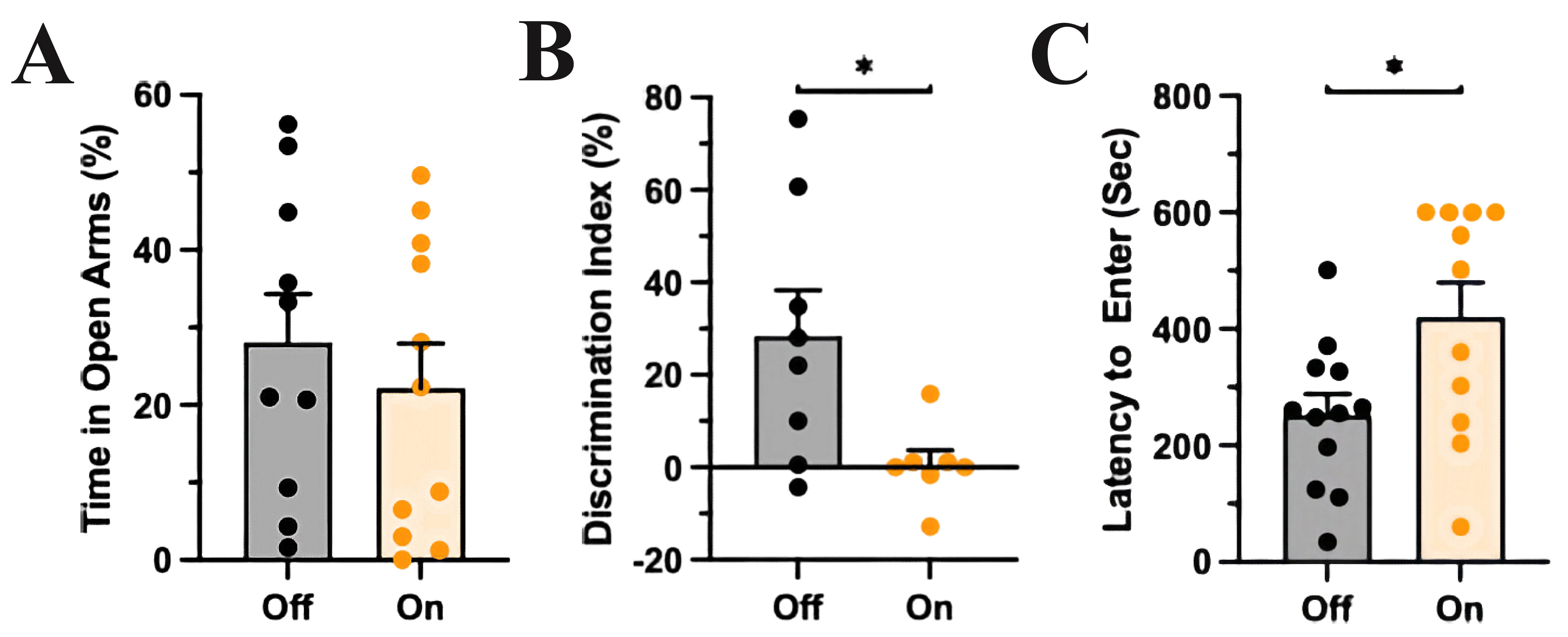
mPFC-LC inhibition did not affect anxiety-like behavior but
impaired recognition memory and problem-solving behavior. (A) The control group
(light off) and the experimental group (light on) spent a similar amount of time
in the open compartments of the EOM, T-test, p
To further investigate the role of the mPFC projections to LC in cognition, we
optogenetically inhibited mPFC terminals in the LC area during the acquisition of
Pavlovian FC. A week after implantation of the bilateral optic fibers, mice in
the experimental group underwent FC with the yellow light emitter on during the
presentation of the CS-US pairing presentation (Box B). The control group
underwent the same treatment in the absence of yellow light. Seven days later,
both groups were placed in a distinct context (Box A) and freezing time was
assessed between groups after presentation of the CS. Both groups spent a similar
amount of time immobile during the acquisition period of the test with the
control group (light off; freezing 12
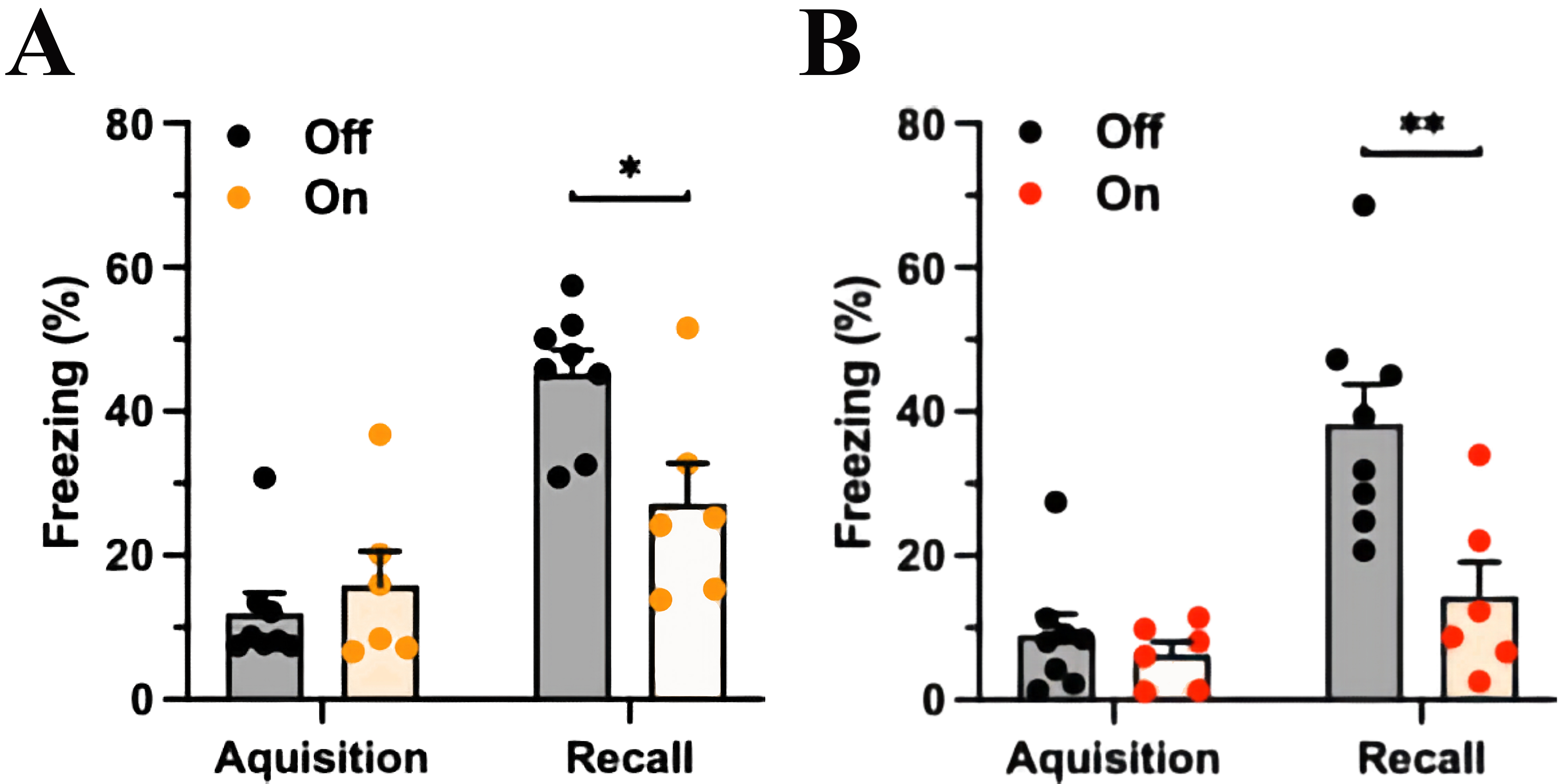
mPFC-LC inhibition during acquisition or during fear recall
similarly reduces CS-elicited freezing. (A) The experimental group (yellow light
on) spent less time freezing in response to the CS than the control group (light
off) with mPFC-LC inhibition during the CS-US pairing at conditioning. The orange
column indicates light exposure, Two-way ANOVA, significant for Fear factor,
p
In another group, the mPFC-LC pathway was manipulated during the testing session
seven days after FC. Analogous to the previous results, both groups spent a
similar percentage of time freezing during the presentation of the CS and US
(control group freezing 8.9
The LC is composed of discrete neuronal subpopulations that deliver NE to
distinct brain regions [7, 32]. In our last experiment, we used anterograde
transsynaptic tagging to trace the anatomical pathways [33] from mPFC to limbic
structures that are functionally involved in cognition. AAV
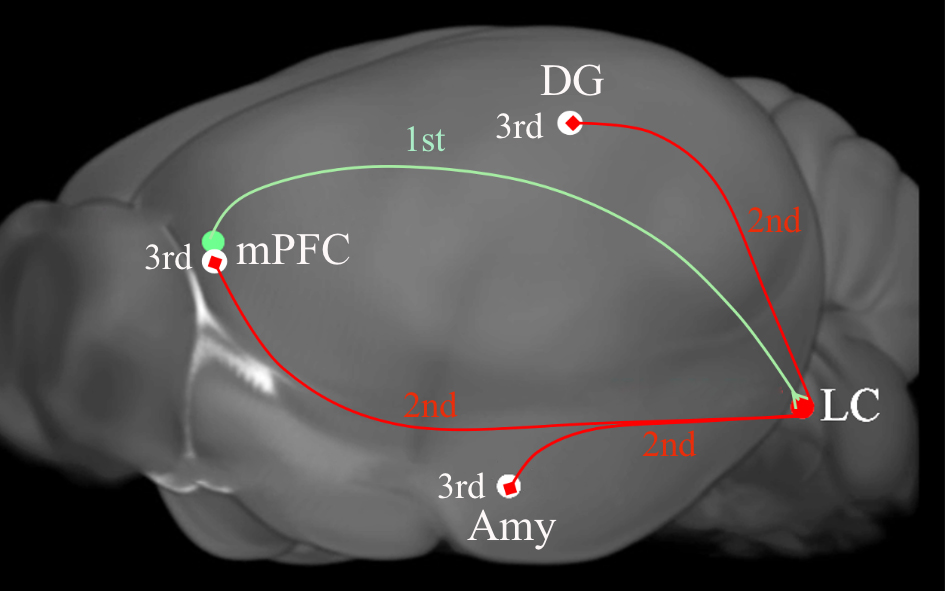
A diagram depicting the tripartite circuits that was revealed by
anterograde transsynaptic cre and flip transfer. Injection of the anterograde
tracer AAV
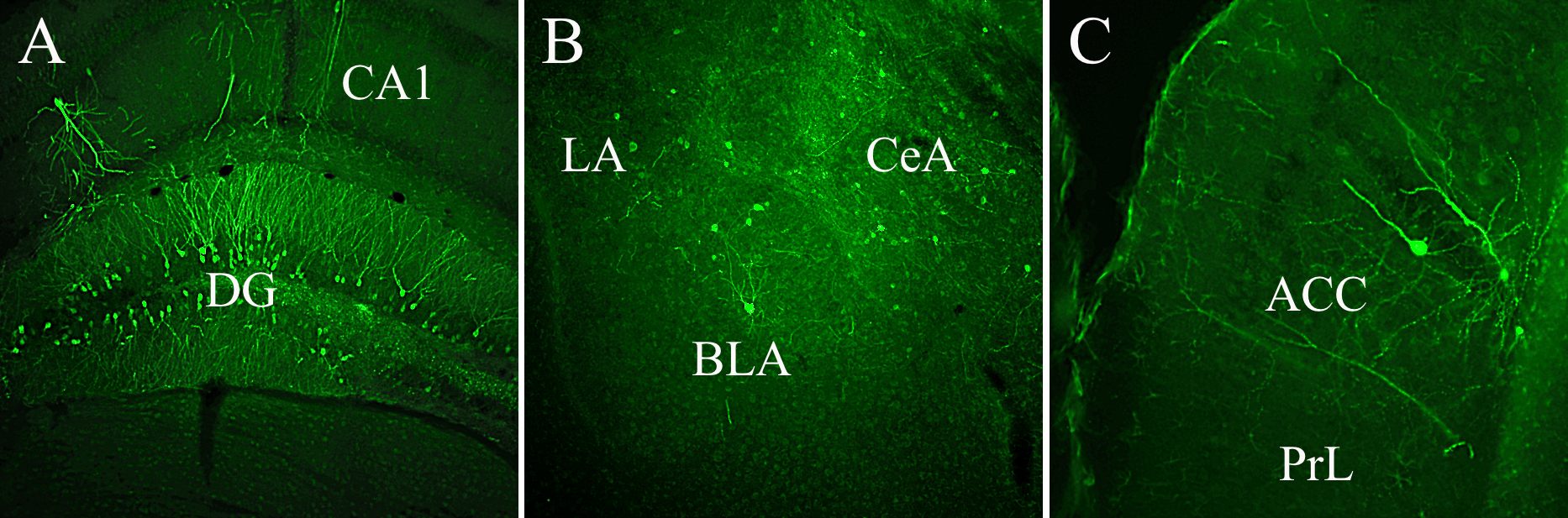
Third-order GFP labeled neurons were expressed by different limbic structures demonstrating continuous, tripartite circuits connecting mPFC to LC and LC to DG, Amy, and mPFC. (A) GFP-labeled cells occupy both blades of the DG, (B) lateral (LA), central (CeA), and basolateral (BLA) subnuclei of the Amy, and (C) the anterior cingulate cortex (ACC). Abbreviations: CA1, field of the hippocampus; PrL, prelimbic cortex; GFP, GCaMP6.
Our results demonstrate direct involvement of the mPFC-LC neuronal projections in learning and memory processes. While optogenetic inhibition of the mPFC efferents in the pontine area of LC did not affect the general anxiety-like behavior, inhibition of the mPFC efferent fibers impaired object recognition memory, problem solving behavior, as well as CS-elicited freezing regardless of mPFC-LC inhibition during FC or testing. The collective behavioral data is supported by the results of our anterograde tracing experiment, which established continuous circuits from mPFC via LC to Amy, DG, and back to mPFC.
LC activity and NE release in the mPFC are pivotal in human cognitive control [34], and primate studies demonstrate an important role for the mPFC in tuning LC activity for optimal performance of a specific task [4]. This mPFC tuning of LC activity is critical, as the literature describes an inverted U-curve relationship between NE release and cognition, where either NE deficiency or excess hinders cognitive functions [4]. The different affinity of mPFC adrenergic receptors for NE is responsible for the dependence of cognitive performance on NE levels [35]. While too much NE release is associated with elevated anxiety levels, low NE levels lead to decreased arousal and attention. The results of our study align with the concept that the mPFC projections to LC are an essential regulatory element for optimal performance in a variety of cognitive tasks.
Pavlovian fear conditioning depends on an interconnected triad of brain structures composed of mPFC, DG, and Amy, where the LC activity and NE release into the triad is central for the acquisition, consolidation, and expression of aversive memories, for review see [36]. Both physical and psychological stressors activate LC with subsequent increased release of NE in Amy [17]. The auditory and nociceptive pathways that convey CS and US information converge onto the Amy and can trigger LTP and synaptogenesis, the basic mechanisms for associative learning, which are supported by optimal NE release [11, 14]. Interestingly, while most studies find that NE release into the Amy is critical for learning and memory processes, some studies find that NE is unnecessary for acquiring and consolidating aversive memories [37, 38]. The general explanation for this discrepancy is insufficient NE release, which strongly depends on stressor severity [39]. Only high-stress levels assure adequate activation of LC, which then delivers optimal NE in the Amy required for the acquisition of the fear association [39]. The selection of a single, high-intensity electric shock in our FC protocol is based the degree of fear expressed in response to the foot shock intensity [40, 41] equivalent to the degree of fear expressed to three weaker shocks [42]. We have shown that this protocol with a single but strong electric shock creates a longlisting fear response in mice [43]. Because LC activation depends on the strength of the foot shock [44], we believe that our single, strong US sufficiently activated LC to levels necessary for emotional augmentation of fear learning. This is supported by a reduction in freezing at test during CS presentations with inhibition of the efferents from the mPFC to LC. These results suggest a critical role for modulation of NE release by the LC via direct mPFC inputs in memory processes.
The initial fear engrams are created in the mPFC, Hipp and Amy but over time, only the engrams in the mPFC and Amy remain active upon recall [22, 45]. The storage of remote fear engrams in the mPFC may explain the results reported here, such that inhibition of the mPFC-LC projections may reduce the effect of fear engrams stored in the mPFC. This would therefore diminish the LC response to CS presentations and the accompanied expression of associative fear.
An important methodological shortcoming of our study is the need for direct evidence for changes in NE release in the targeted structures after inhibition of mPFC-LC neuronal projections. A detailed assessment of NE release in the limbic system after inhibition of the mPFC pontine efferents is our next step in this investigation. For now, the continuous tripartite neuronal circuits between the mPFC, LC, and Amy/DG/mPFC described here provide an anatomical pathway by which the mPFC may regulate NE release in the limbic system. The relatively small number of GFP-labeled neurons in observed in our experiment may be due to few synaptic contacts among targeted cells and NE efferent fibers, where NE released from free axonal endings exert effects via volume transmission [46, 47].
In summary, the study demonstrated the existence of continuous axonal pathways from the mPFC to the Amy, DG, and back to the mPFC via the LC. The mPFC-LC circuit is employed in distinct cognitive processes, such as learning, long-term memory, and problem-solving behavior, where mPFC regulation of LC activity is essential for optimal tuning of the NE release in the execution of memory tasks or solving a problem. In addition, this circuit is likely activated by intense stressors and participates in creating robust aversive memories by mPFC-mediated modulation of LC activity. This work may have far reaching implications for further investigation into the possible role of mPFC-mediated NE release in Post-Traumatic Stress Disorder (PTSD), obsessive ruminations, memory loss, and other cognitive impairments.
In mice, the mPFC projections to LC are essential for optimal performance of cognitive tasks.
All data points generated and/or analyzed during the current study are included in this article and there are no further underlying data necessary to reproduce the results.
ED designed the study, performed the experiments, analyzed and reported the data. The author contributed to editorial changes in the manuscript. The author read and approved the final manuscript. The author had participated sufficiently in the work and agreed to be accountable for all aspects of the work.
All animal work complies with the guidelines set by the IACUC at Rosalind Franklin University of Medicine and Science, protocol number T23-10.
The author is very thankful to Andrea Cardenas for her technical assistance and Nicole Ferrara, PhD, for her editing help.
This research received no external funding.
The author declares no conflict of interest.
Publisher’s Note: IMR Press stays neutral with regard to jurisdictional claims in published maps and institutional affiliations.