- Academic Editor
This article presents evidence indicating that intracranial pressure (ICP) pulsatility, associated with the heartbeat and breathing, is not just a source of mechanical artefact in electrical recordings, but is “sensed” and plays a role in the brain’s information processing. Patch-clamp recording of pressure-activated channels, and detection of Piezo2-protein channel expression in brain neurons, suggest that these channels provide neurons with an intrinsic resonance to ICP pulsatility, which acts to synchronize remote neural networks. Direct measurements in human patients indicate that heartbeat and breathing rhythms generate intracranial forces of tens of millinewtons, exceeding by orders of magnitude the localized forces shown by atomic force microscopy and optical tweezers to activate Piezo channels in isolated neocortical and hippocampal neurons. Additionally, many human touch and proprioceptors, which are also transduced by Piezo channels, show spiking that is phase-locked to heartbeat- and breathing-induced extracranial pressure pulsations. Finally, based on the observation that low-frequency oscillations modulate the phase and amplitude of high-frequency oscillations, body and brain oscillations are proposed to form a single hierarchical system in which the heartbeat is the basic frequency and scaling factor for all other oscillations. Together, these results support the idea that ICP pulsatility may be elemental in modulating the brain’s electrical rhythmicity.
Both the heart and the brain exhibit pressure pulsatility and electrical
rhythmicity. For the heart, the functional link between the two is well
recognized. Electrical rhythmicity drives cardiac-pressure pulsatility and the
pressure-activated channels, Piezo1 and Piezo2, in the aortic arch and carotid
sinus, transduce blood pressure pulsatility to modulate the heart’s electrical
rhythmicity via the baroreflex [1, 2, 3, 4]. For the brain, the boney skull normally
conceals its pulsatility. However, examination of a newborn’s fontanelles or an
adult’s brain either during open-skull surgery, or under
phase-based-motion-amplified magnetic resonance imaging reveals a highly dynamic
pulsatile organ [5]
This review is organized into 10 sections with their topics briefly outlined here. Section 2: Pressure-activated channels in brain neurons. Section 3: Piezo channel gene and protein expression in brain neurons. Section 4: Peripheral baroreceptor transduction of blood pressure pulsations. Section 5: ICP pulse properties. Section 6: The pulsatile sensitivity of pressure-activated and Piezo channels. Section 7: A “proof-of-concept” in the human peripheral nervous system. Section 8: The heartbeat evoked potential and the “pulsatility artifact”. Section 9: The physiology of heartbeat, breathing and brain interactions. Section 10: Cardio-respiratory rhythms linked to electroencephalogram (EEG) recorded brain oscillations. Section 11: Future challenges and in vivo strategies for demonstrating ICP pulsations modulate brain rhythmicity.
As often happens in science [48], several of the key observations that link pressure pulsatility and brain electrical rhythmicity were unanticipated. In 1980, Neher discovered the giga-seal (“tight seal”), which was produced when he applied negative pressure to the pressure port of the patch-pipette holder to draw more membrane into the pipette [49]. Once the sudden and “unexpected” tight membrane-glass seal formed, continuing maintenance of the suction was unnecessary. However, because the seal was mechanically, as well as electrically tight [49, 50], the membrane patch could be stimulated by hydrostatic or osmotic pressure gradients [51, 52]. Indeed, single pressure-activated cation channels were subsequently found to be expressed almost ubiquitously in various vertebrate cell types [53, 54]. More recently, utilizing the thin-slice brain technique and infrared microscopy [55, 56], single pressure-activated cation channel currents were recorded in mouse neocortical and hippocampal pyramidal neurons [16]. Furthermore, the currents displayed channel properties (i.e., cation selectivity, single-channel conductance, inward rectification, and burst gating) like the endogenous pressure-activated cation channels reported in a wide variety of other cell types [51, 57, 58, 59, 60, 61, 62, 63, 64, 65, 66].
A powerful advantage of the cell-attached patch technique is that it allows
simultaneous, “non-invasive” monitoring of spike activity in the whole cell
[67]. In particular, the membrane patch capacitance acts to differentiate the
action potential waveform, generating a typical, pronounced, outward current
spike in the membrane patch. This technique has been used to record spike
activity in several types of neurons [68, 69, 70, 71] and confirm the sparse firing of
neocortical pyramidal neurons in the anesthetized and awake mammalian brains
[13, 72]. Fig. 1A,B (Ref. [16]) show cell-attached patch recordings from a
hippocampal pyramidal neuron in which brief (
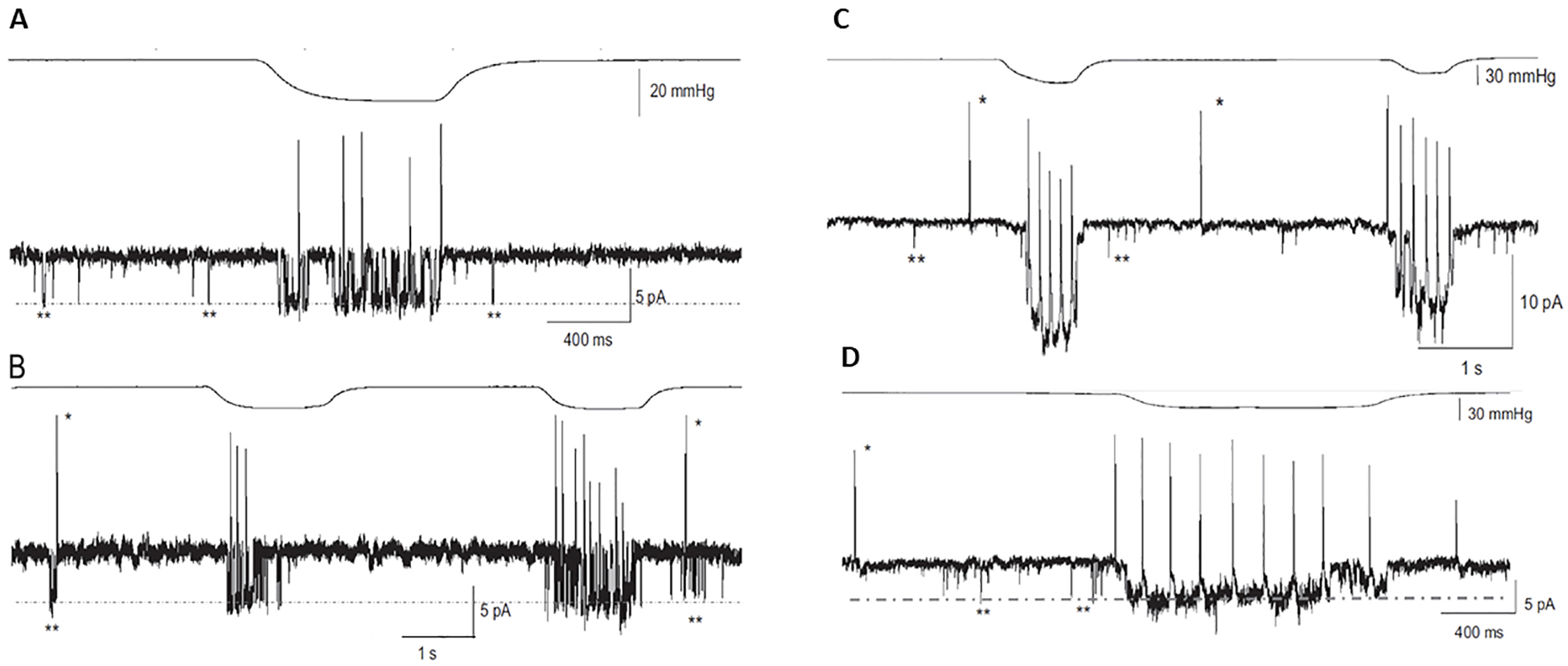
Pressure-activated single channel currents trigger
spiking in mouse hippocampal and neocortical pyramidal neurons. The top traces
in (A–D) show the applied negative pressure pulse waveforms. (A) The lower trace
is the cell-attached patch-current recorded from a mouse hippocampal pyramidal
neuron indicating “spontaneous” brief (
Previously, resting or “basal” activity of single pressure-gated channels, as
seen in Fig. 1, has been proposed to arise from a significant resting tension
generated by the membrane patch being “pulled flat” by tight-seal formation
[73, 74, 75, 76]. However, in the specific case of pyramidal neurons localized within the
highly folded cortex, tension already exists as indicated by a rapid recoil of
cut axon ends [77, 78, 79, 80]. Moreover, vertebrate neurons, unlike many other cell types
(see [74, 75]) do not express microvilli or caveolae that would, by providing
excess membrane, minimize any sustained membrane tension [81, 82, 83]. Given these
features, basal channel activity in pyramidal neurons may provide an added source
of membrane channel noise [84] contributing to the stochastic resonance proposed
to occur in neocortical and hippocampal neurons [85, 86]. In addition, ICP
fluctuations (
The membrane protein that forms the endogenous pressure-activated cation channel
was discovered in 2010 by Patapoutian and colleagues, using a short interfering
RNA knock-out screen to identify a novel membrane protein-channel family, which
they designated Piezo [17]. Vertebrates express two family members, Piezo1 and
Piezo2, shown by reverse transcription-polymerase chain reaction to be
differentially expressed in various mouse tissues, including brain [17].
Significantly, cell-attached patch recordings have indicated that the single
Piezo1 channel has a monovalent cation conductance of ~60 pS,
like the ~60 pS channel measured in cerebral pyramidal neurons
under similar divalent-free ionic conditions [16, 89]. Moreover, a in
situ hybridization study [18], reported that PIEZO1—previously identified as a gene transcriptionally upregulated in astrocytes by
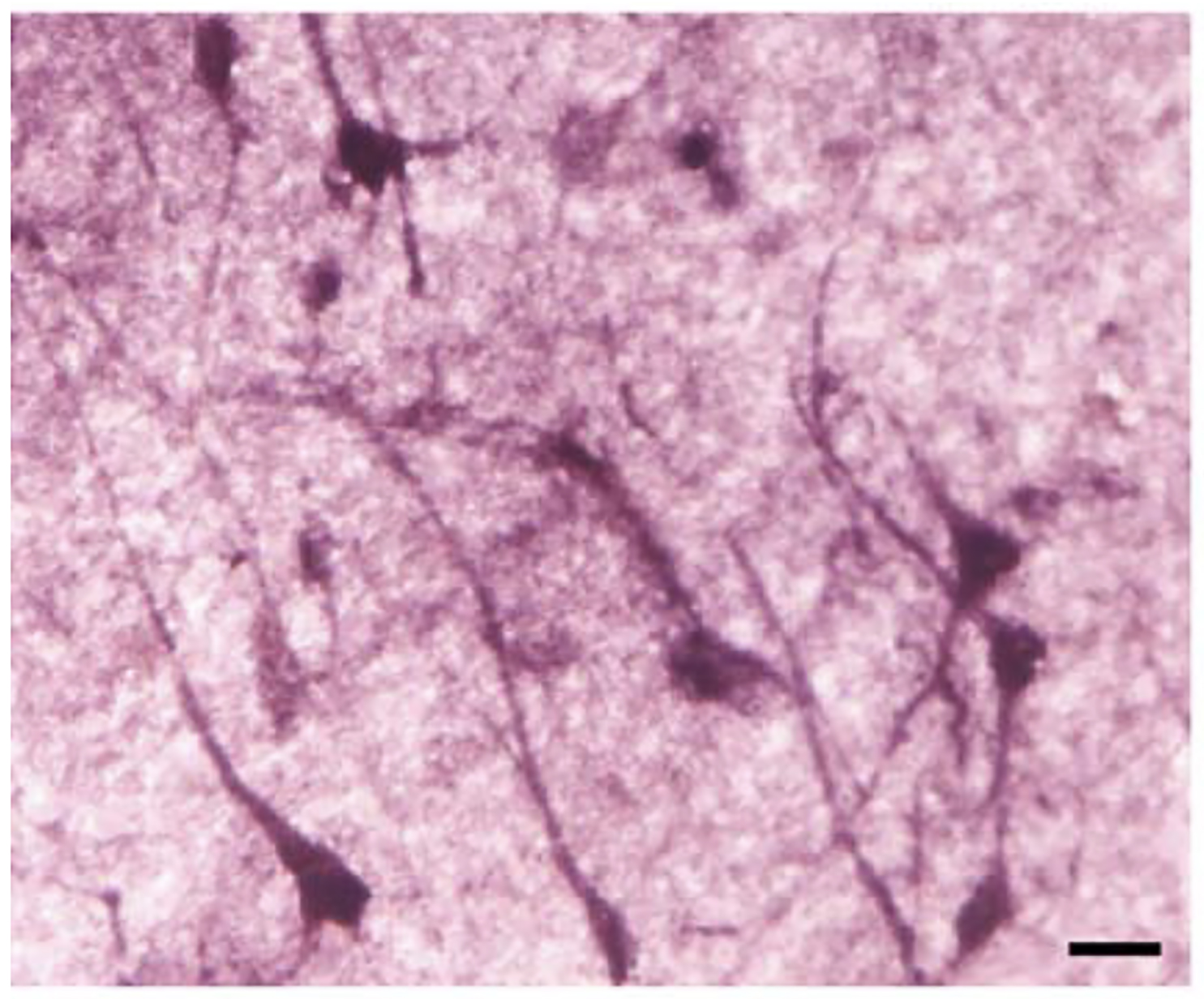
PIEZO1/MIB (Membrane protein induced by
Immunohistochemistry (IHC) has confirmed Piezo2 channel-protein expression in
mouse neocortical neurons (particularly in pyramidal neurons of layers 5 and 6),
hippocampal pyramidal neurons (particularly in the CA3 region), and Purkinje
cells of the cerebellar cortex [19]. Moreover, human IHC studies by the Human
Protein Atlas (HPA) group, using a different anti-PIEZO2 antibody, found PIEZO2
expression in neocortical and hippocampal neurons, as well as selective
expression in cerebellar Purkinje cells.
The Piezo2 protein expression in Purkinje cells did not express as single
pressure-activated channels or pressure-induced alterations in their rhythmic
spiking, even when the negative pressure (suction) pulses were increased to
levels that ultimately caused patch rupture (
A conceptually important and unanticipated IHC result was the selective Piezo2
expression in mitral cells of the mouse olfactory bulb (OB) (Fig. 3, Ref. [19]).
At the same time, a single nucleus RNA sequencing study, also indicated
Piezo2 as a genetic marker of mouse OB mitral cells [93], and
transcriptomic data on the HPA website described Piezo2, as well as
Piezo1, expression in the OB of human, pig, and mouse
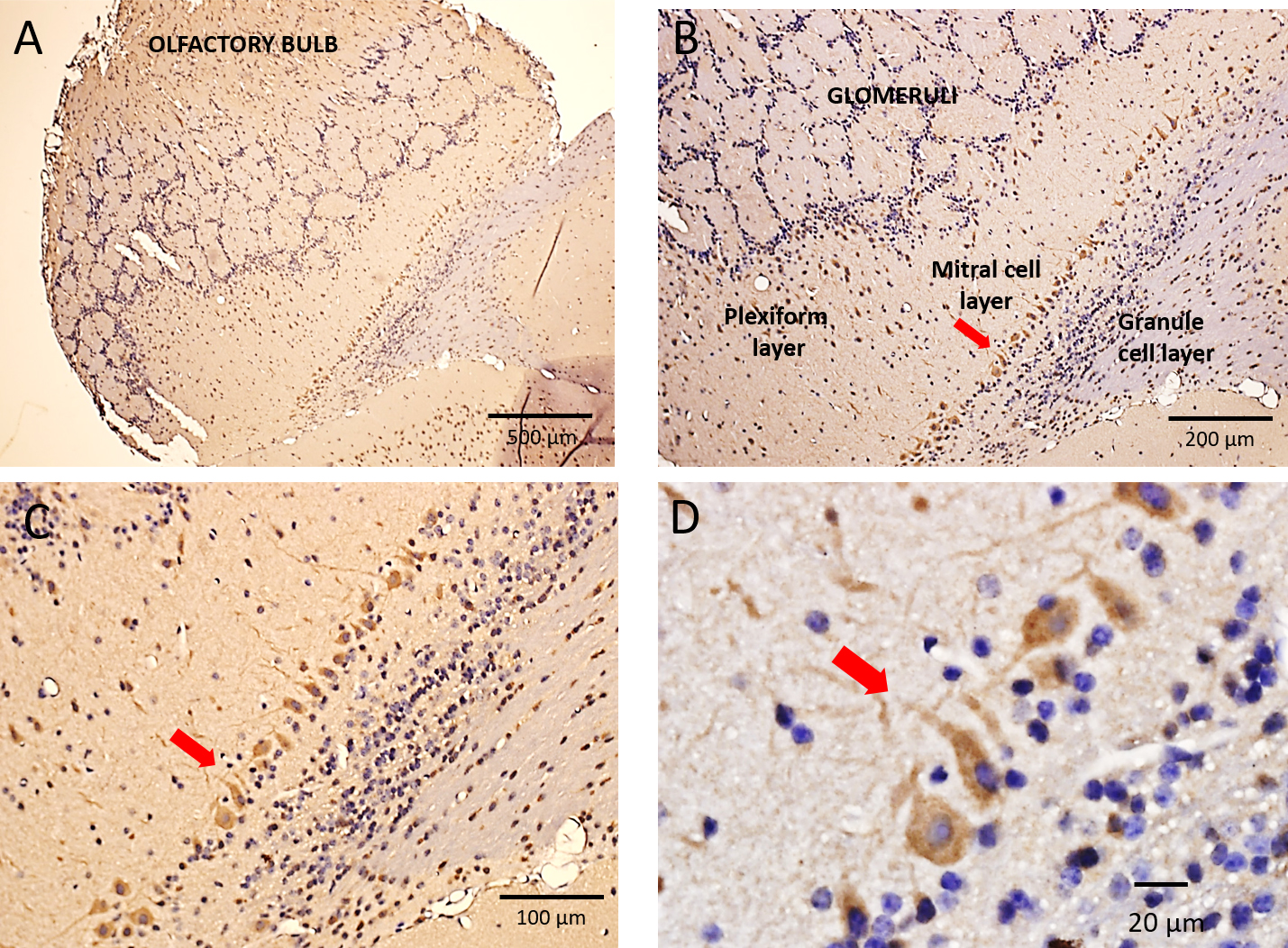
Immunohistochemical localization of Piezo2 in the mouse
olfactory bulb. (A) A low magnification image of the mouse olfactory bulb (OB)
with its characteristic circular/spherical glomeruli structures spanning the OB.
These glomeruli include the synaptic connections formed between primary olfactory
nerve axons and mitral cell dendrites. (B,C) Higher magnification images
(10
Piezo channels are involved in a wide variety of peripheral mechanosensory functions, including somatosensation [17, 28], proprioception [29, 97, 98], breathing [99] and blood pressure regulation [3]. Of particular interest here is their role in the regulation of blood pressure and heart rate [3, 100]. The baroreceptor neurons that innervate the aortic arch and carotid sinus rapidly transduce (via Piezo1 and Piezo2) the beat-to-beat changes in blood pressure, and then, through the baroreflex, involving the vagal nerve-brain stem loop, regulate heartbeat and blood pressure [1, 2, 3, 4]. Direct support for this role is that optogenetic activation of Piezo2 in baroreceptor neurons decreases heart rate and blood pressure, consistent with baroreflex activation, whereas genetic deletion of Piezo1 and Piezo2 in the neurons abolishes the baroreflex [3]. A more recent study indicates that selective deletion of Piezo2 alone in baroreceptor neurons also eliminates the baroreflex [4]. Moreover, morphological analysis of the same neurons has indicated they form macroscopic claws that exude fine end-net endings that surround the aortic arch. This provides structural insight into how blood pressure is sensed in the arterial wall [4].
Mean arterial blood systolic and diastolic pressures are typically ~120 mmHg and ~80 mmHg, respectively, so with each heartbeat, there is also a ~40 mmHg pressure pulse of ~1 s duration (Fig. 4A, see [1]). Studies of single baroreceptor unit activity indicate a much lower threshold (i.e., by ~30 mmHg) for pulsatile than for static pressures [1]. In addition, dynamic pressure stimulation and pulsatile activity were shown to better augment the baroreflex [100, 101]. The question then is whether the extrinsic properties of the ancillary structures (i.e., claws and fine end-net endings) or the intrinsic gating properties of the pressure activated channels (or both) determine the higher sensitivity of baroreceptors to pulsatile vs. static pressures [1, 4]? This is relevant for brain neurons that express Piezo channels and presumably lack the specialized ancillary features of baroreceptor neurons, but as described next, are also exposed to pulsatile as well as steady-state ICP.
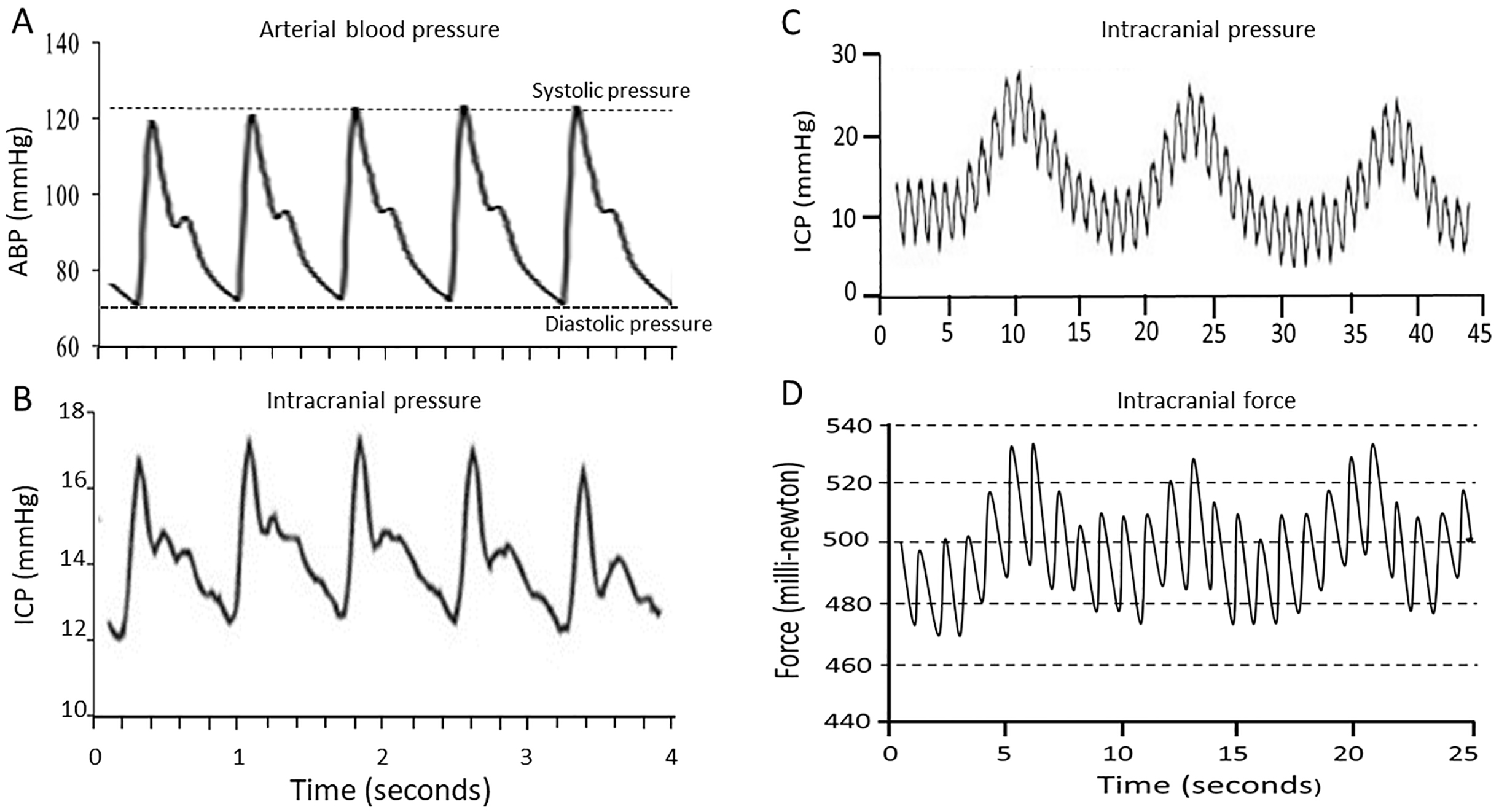
Arterial pressure and intracranial pressure/force pulsatile recordings. (A) Typical arterial pressure pulse waveforms of ~40 mmHg amplitude. (B) Typical ICP pulse waveforms of ~4 mmHg amplitude measured over a short time interval of 4 s. (C) Typical ICP pulse waveforms measured over a longer time interval of 45 s indicating a fast pulse of a ~1 s that was synchronized with the heartbeat and a slower pulse of ~10 s synchronized with respiration. (D) Pulsatile intracranial force measured using implanted force transducers indicate two pulse waveforms of ~1 s and ~10 s. Data from Ref. [21] was used to generate the graph with permission of the authors: Goldberg CS, Antonyshyn O, Midha R, Fialkov JA. Measuring pulsatile forces on the human cranium. Journal of Craniofacial Surgery. 16, 134–139, (2005). ICP, intracranial pressure.
The rigid cranium underlies the brain’s extremely low compliance and limited
capacity to increase in volume in response to influx of arterial blood.
Consequently, brain perfusion which is a function of cerebral perfusion pressure
(CPP) is determined by the difference between mean arterial pressure (MAP) and
the opposing ICP (i.e., CPP = MAP – ICP). It is this relationship that motivated
the development of techniques to monitor ICP in patients suffering from brain
swelling due to traumatic brain injury, hydrocephalus, or cerebral hemorrhage.
For example, ICP directly measured by insertion of pressure transducers into
either the ventricles or the brain parenchyma, should normally show low basal
levels (i.e.,
In addition to displaying mean baseline values, ICP also undergoes
heartbeat-related pulsations similar in waveform and duration (i.e.,
~1 s) to arterial blood pulsations, but with lower amplitude
(i.e.,
Other studies have used non-invasive techniques—transcranial doppler
ultrasound and phase contrast magnetic resonance imaging—to measure
the pulsatile nature of CSF flow and brain motions [5, 104, 119, 120]. However,
there appears to be only one study that has directly measured the pulsatile
forces that generate these different pulsatile phenomena. Goldberg and colleagues
[21], by inserting a force transducer into the epidural space at the periphery of
a craniotomy performed on neurosurgical patients, measured two pulse waveforms—a ~1 s duration, ~30 mN pulse and a
~10 s, ~20 mN pulse—synchronized
with the patient’s heartbeat and ventilation rate, respectively (Fig. 4D, Ref. [21]).
Given these forces plus the reported surface area of the transducer (9
Another important issue regarding ICP pulsatility relates to the mechanism(s) that generate and transmit the ICP pulse throughout the brain. This has relevance because it is this ICP pulse transmission that has been proposed to rapidly synchronize remote neural networks [19]. During systole the arterial-blood inflow to the brain transiently exceeds the venous outflow, so that the brain experiences a transient expansion in volume. It is this volume increase that generates the ICP pulse [122]. However, the exact mechanism by which the ICP pulse is transmitted throughout the brain remains unresolved. One theory, referred to as the “acoustic transmission theory”, assumes that the ICP pulse represents the arterial pressure pulse, and this is what is propagated throughout the CSF space as a traveling (or transmitted) wave, at the speed of sound in water (i.e., ~1500 m/s). In this case, the ICP pulse should be detectable almost instantaneously throughout the brain and should be synchronized with the arterial pulse [123, 124]. In contrast, what is referred to as the “resonance theory” assumes a cerebral Windkessel effect prevents the direct spread of the arterial pulse (i.e., as a bolus of blood) throughout the rest of the vasculature (i.e., capillaries and veins) [125, 126, 127]. Instead, during systole the CSF links the arterial radial expansion to venous compression, and then during diastole the CSF links venous expansion to arterial relaxation. In this way, two travelling waves are created—one wave excited by an external force and another reflected by the elastic contents of the cavity—that are superimposed to create a standing wave that oscillates rather than travels (Fig. 5). It is also this CSF-mediated reciprocal, equal and opposite, venous compression and expansion that acts as a pulse absorber to protect the delicate cerebral capillaries from pulsatile forces [127]. In this respect, the cerebral Windkessel effect differs from the peripheral Windkessel effect, in which the aorta acts as an elastic buffering chamber to transiently store ~50% of the systolic stroke volume and then push it forward during diastole to create a continuous blood flow [128]. However, because of the high compliance of peripheral tissue, the elastic energy of each pulsatile aortic expansion is dissipated throughout its surroundings. This mechanism of energy dissipation cannot occur in the brain because of its extremely low compliance (i.e., each heartbeat generates only a ~1 mL expansion, or ~0.08% assuming a ~1300 mL brain volume [122]). Instead, the cerebral Windkessel’s two-way arterial-CSF-venous pump generates both resonant and anti-resonant properties, ensuring efficient perfusion and capillary protection, respectively [127].
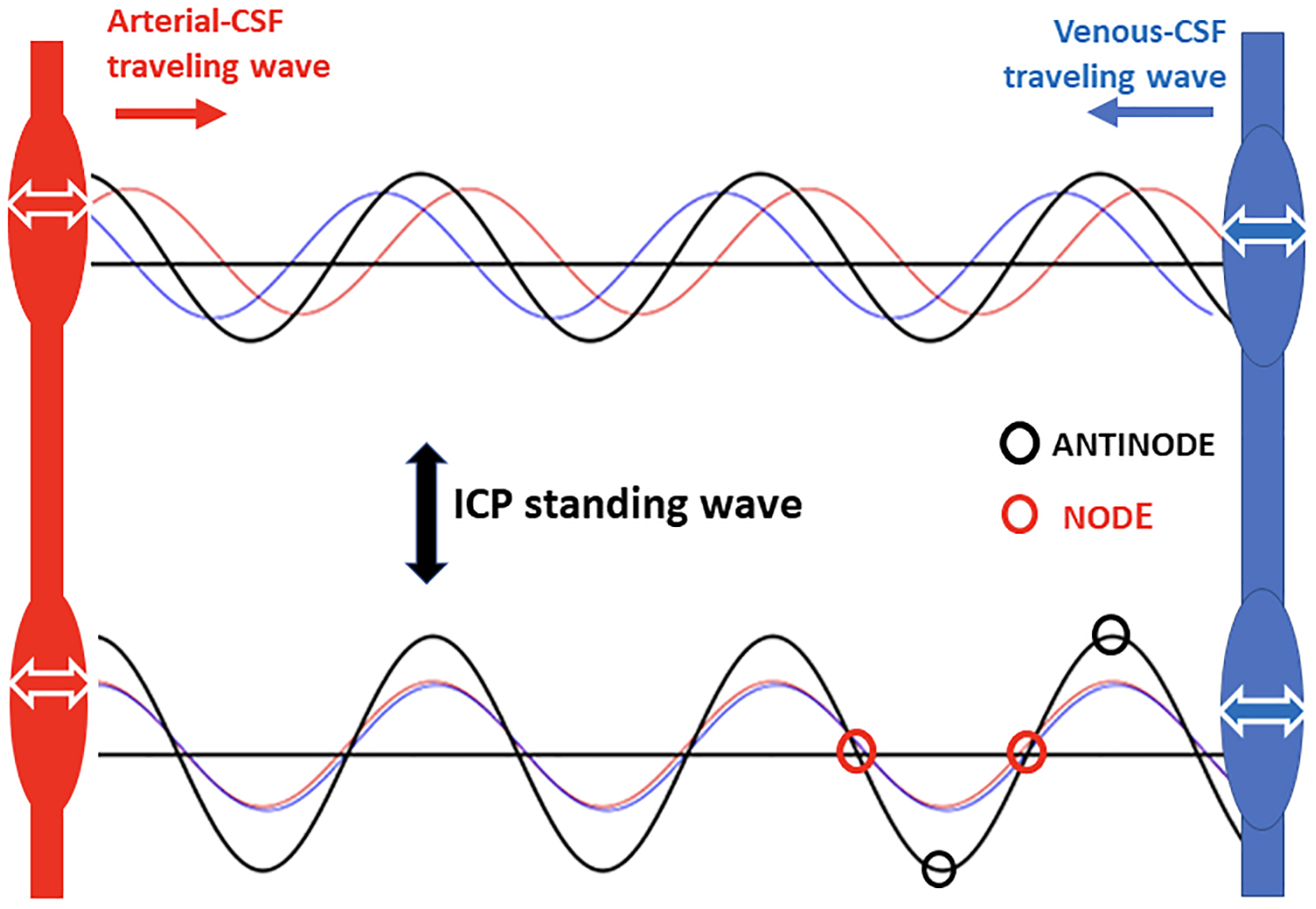
Schematic representation of the ICP standing wave generated by the superposition of two traveling waves. One generated by the arterial pressure pulse perturbation of the cerebrospinal fluid (CSF) and the other a reflected travelling wave generated by the venous recoil that perturbs the CSF. The key difference with the standing wave is that it oscillates up and down without travelling and this way generate antinodes of localized high pressure and nodes of localized minimal pressure.
The resonance theory, not yet universally accepted [123, 124], can account for several key experimental observations [127]. First, the unexpected observation that the ICP pulse can precede the arterial pulse would seem to rule out a simple transmission theory but can be explained if the brain has its own resonance properties that filter fast-frequency components of the arterial pulse, thereby creating asynchrony with the ICP pulse [127, 129]. Second, at heartbeat frequency there is a low amplitude component of the ICP pulse, referred to as a “notch”, which is consistent with anti-resonant behavior, and which disappears during either intracranial hypo- or hypertension, presumably because the anti-resonant effect is lost under both abnormal conditions [127, 129]. The resonance theory raises several questions in relation to possible ICP pulse synchronization of neural networks. First, does the standing wave collapse between cardiac pulses, and if so, what are the consequences? This would seem particularly important when pulse frequency is significantly slowed, perhaps most dramatically during freediving in humans to ~10 beats per minute [130, 131]. Second, since standing waves are characterized by maximal and minimal pressures, referred to as antinodes and nodes, respectively (Fig. 5), do privileged regions or network hubs exist within the brain that are subjected to specific pressure domains?
Given the forces and dynamics of ICP pulsatility discussed above, the question
is whether pressure-activated and Piezo channels possess the dynamic force
sensitivity to transduce ICP pulsations efficiently? The development of the fast
pressure-clamp enabled measurement of the rapid kinetics of single
mechanosensitive channels [132, 133, 134]. Introduced before Piezo identification, it
was first used to analyze the gating of the endogenously expressed
pressure-activated cation channels in various cell types
[133, 135, 136]. Fig. 6A (Ref. [75, 133]) shows the transient
response to stepwise increases in pressure with rapid (i.e.,
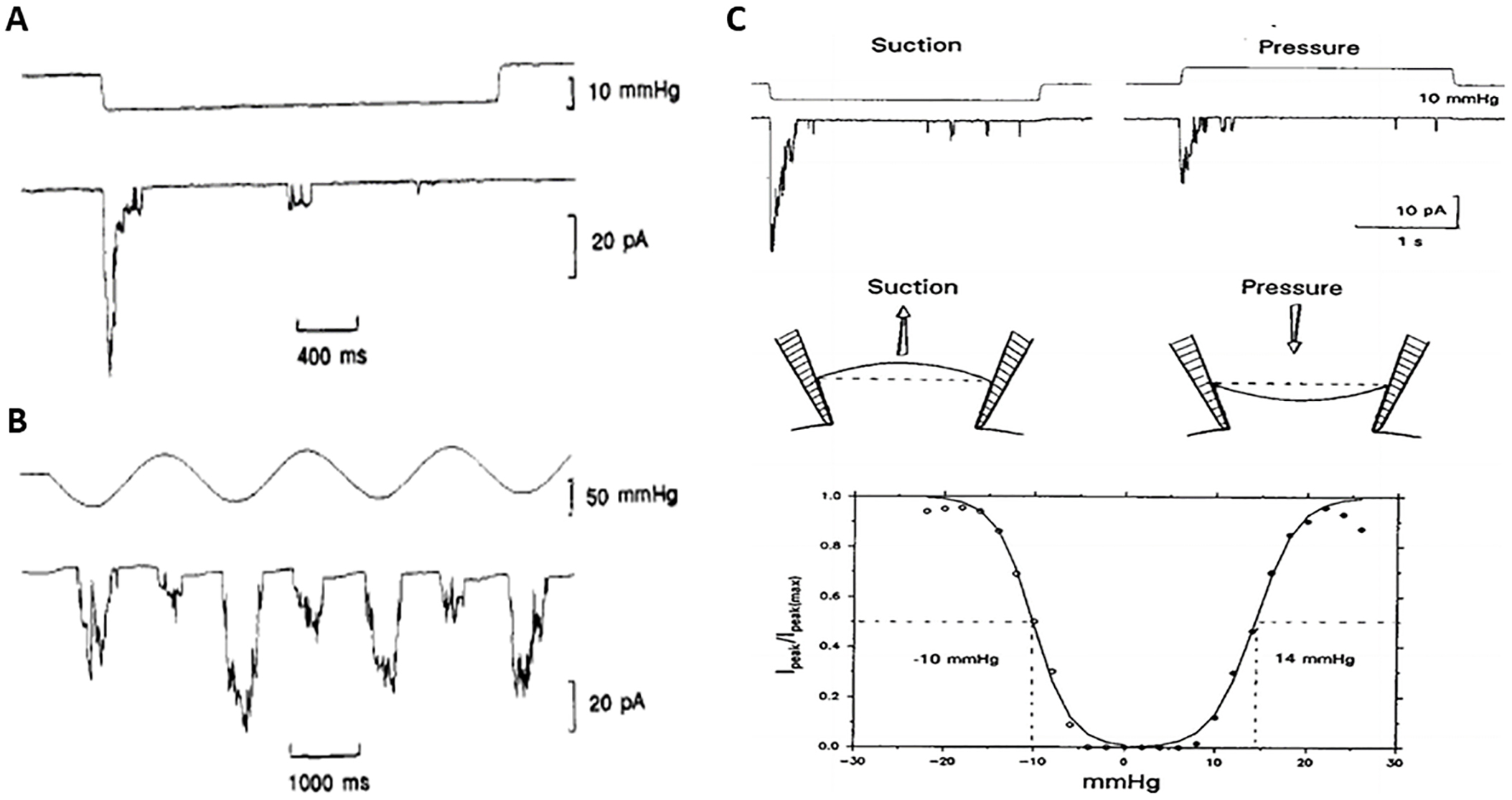
Gating kinetics and pressure sensitivity of endogenously
expressed pressure-activated cation channels in Xenopus oocytes. (A)
The upper trace is the pressure step waveform (2.5 s) applied to a cell-attached
patch. The lower trace is the activated channel current showing rapid channel
opening (
Estimates of pressure/force sensitivity vary with specific measuring techniques
and recording conditions. Cell-attached patch recording combined with a
gentle-sealing protocol [135, 136], can yield maximum sensitivity, which is
otherwise lost with “hard seals”, overstimulation of the patch, or by membrane
blebbing [135, 136, 140]. For example, in the patch described in Fig. 6C [75],
obtained with a gentle seal, the pressure-current relations in response to brief
step changes in negative and positive pressures indicated that pressures that
activated half the channels (P
Two recent studies have specifically measured the pressure/force required to
stimulate neocortical and hippocampal neurons that were grown in tissue culture
[24, 26]. In one case, an oscillating fluid shear stress of only 1–5 Pa
(0.0075–0.038 mmHg) activated intracellular Ca
In the same study [24], but using atomic force microscopy (AFM) to apply a
highly localized indentation force of 100 nN to the neuron soma, global
[Ca
In the study that focused on cultured hippocampal neurons [26], an oscillating
optical trap that indented the neuron with forces as low as 13 pN, activated
[Ca
Touch receptors in the skin are highly specialized to transmit information to
the central nervous system (CNS) about the forces exerted by the “tactile
world”, whereas stretch receptors located within intrafusal muscle fibers are
specialized to transmit information on the exact position and movement of the
body in space. However, Macefield and colleagues [30, 31] have also shown that
both mechanoreceptors respond to the local pressure pulsations associated with
heart and breathing rhythms. Specifically, the spike discharge of many human
fingertip touch receptors (~50%) and human muscle stretch
mechanoreceptors (~60%) are either phase locked or modulated by
the arterial pressure pulsations generated within the local vascularized tissue
[30, 31]. Fig. 7 (Ref. [31]) shows recordings from a human muscle spindle that
indicate that a spike is activated with each heartbeat in the absence of any
other spontaneous spike activity [31]. Moreover, the authors also found that the
discharge of a smaller proportion (10%) of spindle afferents displayed
respiratory modulation ([31], see also [149, 150]). The exact
role, if any, of this physiological noise that is transmitted to the CNS via the
somatosensory and propriosensory neural pathways, remains unclear. Perhaps the
noise is filtered out or ignored by the CNS as an interference during perception.
However, arterial pulsations by acting to synchronize a barrage of afferent
incoming spikes within a narrow time window (i.e.,
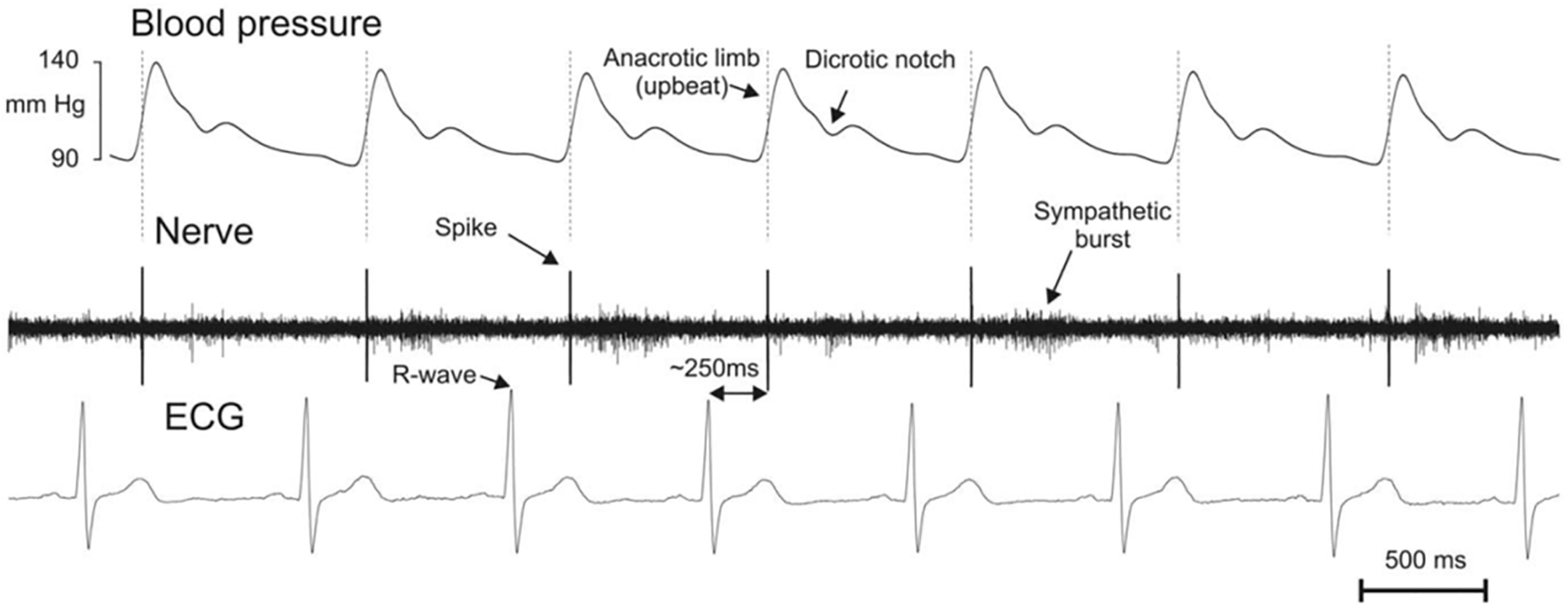
Example of muscle spindle discharge locked to the arterial pressure pulsations. This afferent responded with one single spike at the early part of the upbeat of pulse wave ~250 ms following the R-peak in the electrocardiogram (ECG) signal. Note the absence of spontaneous spike activity but the indicated presence muscle sympathetic burst activity. Reproduced from Ref. [31] PLoS ONE, Birznieks I, Boonstra TW, Macefield VG. “Modulation of Human Muscle Spindle Discharge by Arterial Pulsations - Functional Effects and Consequences”. 7(4), e35091, (2012) with permission from John Wiley and Sons.
Several key features of peripheral mechanoreceptor spike entrainment by
cardio-respiratory rhythms [30, 31] have provided “proof of concept” that
Piezo2-dependent cardio-respiratory entrainment may also occur in brain neurons
[19]. First, Piezo2 channels underlie mechanotransduction in both touch [17, 28]
and muscle stretch receptors [29, 97, 98]. Second, the arterial pulsatile force
that activates Piezo2 in the human fingertip pad was measured at
~6 mN [30], which is significantly smaller than the measured
cardio-respiratory-induced intracranial pulsatile forces of 20–30 mN [21].
Third, like the human finger pad [157] the human brain is highly vascularized
with most neurons located close to (
The heartbeat evoked potential (HEP) is measured by averaging brief time segments of scalp electroencephalogram (sEEG) or intracranial EEG (iEEG) recordings, time-locked to the heartbeat, usually the R peak of the electrocardiogram [14, 15, 32]. The neural pathways transmitting the HEP to the brain begin with a variety of Piezo-dependent mechanoreceptors that sense pulsatile changes in the heart, arteries, skeletal muscle, and skin of the chest wall [161]. The afferent output of the mechanoreceptors is transmitted mainly by the vagus nerve [162], but also by glossopharyngeal and spinal nerves, to the brainstem and thalamus; from there they are sent to higher brain regions including the central autonomic network (i.e., insula, amygdala, anterior cingulate and hypothalamus) and somatosensory cortices [163, 164, 165] with the HEP arriving 50–550 ms after each heartbeat [166, 167, 168]. The central autonomic network is notable for its control over preganglionic sympathetic and parasympathetic motoneurons [163].
The HEP, although similar in several ways to other sensory evoked potentials, is also significantly different. First, for the brain the HEP is ever-present, from gestation to death, and therefore must be considered a constant component of the brain’s intrinsic electrical activity. Second, the HEP does not perform a sensory role, in that the person is usually not conscious of the heartbeat. Instead, because the HEP impacts widely spaced neural networks [163, 164, 165, 166, 167, 168], it functions more in creating a “global moment” involving perception, emotion, cognition, and self-consciousness [33, 34, 35, 36]. Indeed, changes in psychological properties, including attention, emotion, empathy, and cognition are reflected in modulation of the recorded HEP [168]. Therefore, the HEP does not function to convey cardiac-related information to the brain, but rather to modulate specific brain-network activity and functional connectivity [35]. For example, the HEP response is not generated by a simple summing of individual HEP responses, but rather by promoting phase-resetting of ongoing intrinsic neural activities [14, 15].
Another feature of the HEP, and most relevant for this discussion, is the so
called “pulsatility artifact” that is typically seen as a HEP contaminant by
causing mechanical displacement of electrodes to alter their impedance and
recorded HEP [14]. Consistent with this idea, is that the pulsatility artifact is
much larger (i.e., ~6
Breathing and heartbeat, as the two major oscillatory rhythms of the body,
continuously interact with each other and with the brain [32, 33, 34, 35, 36, 37, 38, 39, 40, 41, 42, 43, 171]. Breathing,
unlike the heartbeat, has no intrinsic pacemaker and stops when disconnected from
its central input. Also, unlike the heartbeat, breathing frequency and depth of
breathing are subject to rapid and conscious alteration. However, normal
breathing is also a major and constant modulator of the intervals between
heartbeats (i.e., Heart rate variability (HRV)) in which HR increases with
inspiration and decreases with expiration [171, 172, 173, 174, 175, 176, 177]. This process has been
proposed to maximize respiratory gas exchange [175] and is a form of HRV,
specifically referred to as respiratory sinus arrhythmia (RSA), in which the
maximum differences in HR (HR
The functional implication of HRV is that the heartbeat is not a metronome [173]. Instead, a low HRV is seen as a sign of poor health and vulnerability to physical/psychological stressors and disease [178], whereas a high HRV is associated with emotional resilience, cognitive flexibility, and a more developed capacity to control affective, cognitive, and physiological aspects of stress [35, 171, 172, 173]. Obviously, a high HRV provides the healthy heart with the potential to respond to physical and anticipatory demands. However, it is not so obvious why or how a high HRV translates into superior behavioral responses. This question may best be addressed in terms of resonant breathing, which maximizes RSA/HRV by reducing the breathing frequency from the normal 12–18 breaths/min (0.2–0.3 Hz) to a slow ~6 breaths/min (~0.1 Hz) [179, 180]. In this case, the slow breathing of ~10 s per breath has the same duration as the baroreflex loop that includes ~5 s to transmit blood pressure changes to the brain, and ~5 s to transmit the output back, to alter HR and blood pressure. Consequently, during resonant breathing the baroreflex, blood pressure and respiration are all coordinated and combine to generate an HRV response that far exceeds that predicted from simple additive effects (i.e., they resonate). Fig. 8 (Ref. [35]) illustrates this dramatic resonant effect involving large (~20 mmHg) sinusoidal oscillations in HRV. Specific subjects that naturally display a high HRV show increased blood flow to brain regions involved in executive and emotional functions including the prefrontal cortex and the amygdala [35]. Therefore, one possible explanation is that the same brain regions determining improved brain functions also determine HRV, in which case high HRV may simply be a peripheral “indicator” of central functions. However, evidence against this pure indicator role is that when a high HRV is induced by resonant-breathing-biofeedback sessions, it relieves anxiety symptoms in patients suffering from depression or posttraumatic stress disorder and improves motor performance and cognitive flexibility in normal subjects [35, 168, 172, 173]. Based on these observations, Mather and Thayer [35] proposed that resonant breathing promotes functional connectivity in those brain regions. In which case, several non-exclusive mechanisms as listed below may be involved:
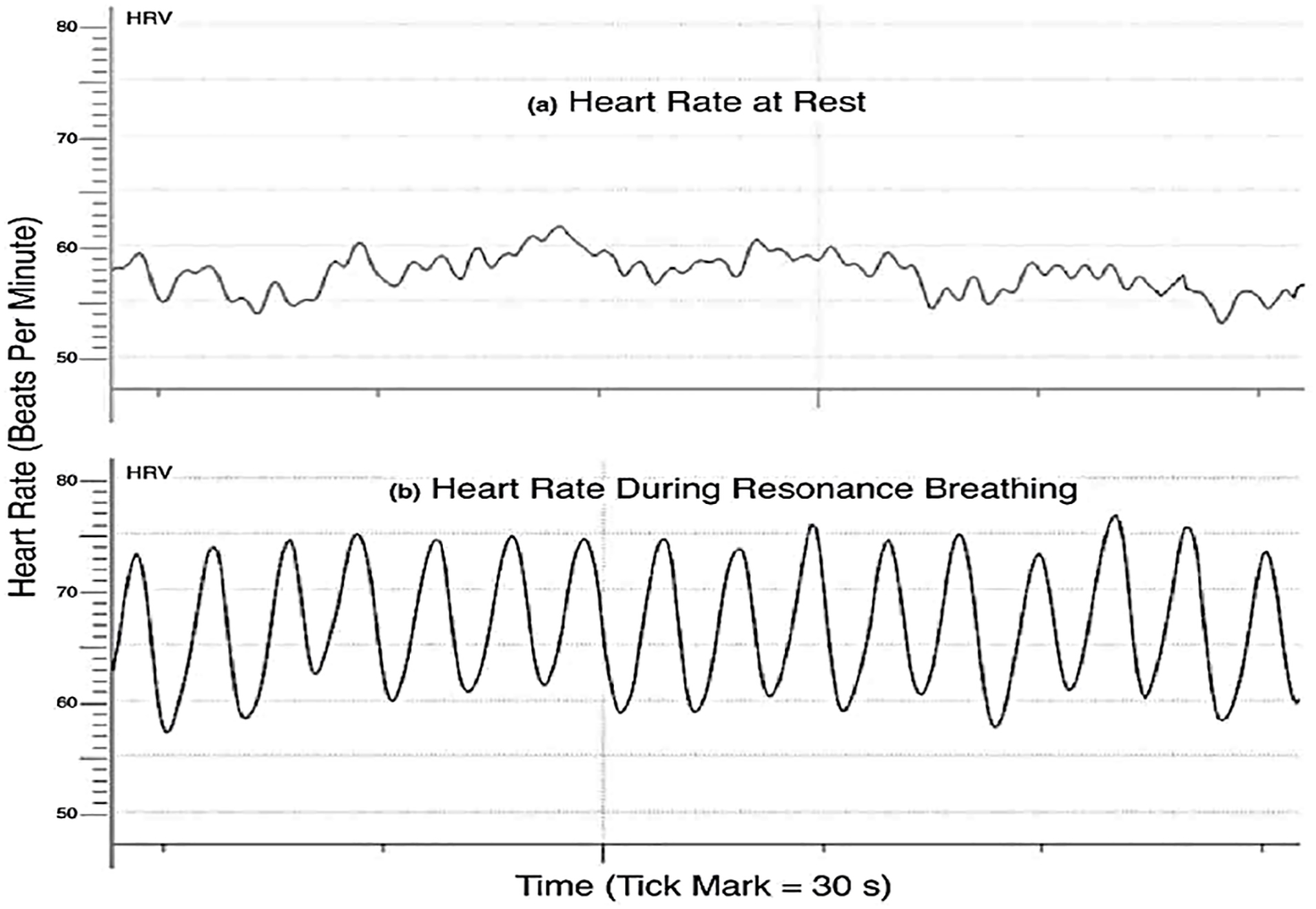
Heart rate (Beats Per Minute) during normal and resonance breathing. (a) An example of heart rate variability during about a 2.5 min period during quiet rest. (b) The same person’s heart rate during resonance breathing during another 2.5 min period. Reproduced from Ref. [35] Current Opinion in Behavioral Sciences. Mather M, and Thayer J. “How heart rate variability affects emotion regulation brain networks”. 19, 98–104, (2018) with permission from Elsevier.
(i) Increased blood-oxygen supply promoted by resonant breathing, as measured by fMRI, increases functional connectivity among specific brain regions involved in cognition and emotion [181].
(ii) Heartbeat-generated HEP (accompanied by the so-called pulsatility artifact) that resets intrinsic brain oscillations in autonomic brain regions involved in regulating emotion and cognition is increased in amplitude by resonant breathing [168, 173].
(iii) Increased gain in the baroreflex that interacts bidirectionally with brainstem and forebrain regions, including those in the central autonomic network [163], that regulate arousal and parasympathetic vagal tone, specifically contributing to the anxiety-reducing effects of resonant breathing [35].
(iv) Breathing itself, particularly slow-paced and deep-nasal breathing, which has been recognized as entraining electrical oscillations in neural networks due to olfactory reafferent discharge (see below), most notably in limbic and prefrontal cortical regions [38, 39, 40, 41, 42, 43].
(v) Resonant breathing by increasing the amplitude or duration of the cardio- and respiratory-related ICP pulsations [114, 115, 116, 117, 118] enhances (via Piezo2/ICP transduction) entrainment and functional connectivity of neural networks [19].
Regarding the last mechanism, a general synchronization and feedforward coherence has been reported between beat-to-beat ICP changes and HRV [182] and interpreted as ICP effects on the central autonomic network that regulates HRV [183]. However, whether the Piezo/ICP and/or the other mechanisms are elemental to this regulation, remains to be determined.
A remaining issue concerns how the heartbeat (~1.25 Hz) and
respiratory rhythms (~0.25 Hz) relate to traditional brain
oscillations measured by EEG (
Using a different approach and based on the observation that low frequency brain
oscillations tend to modulate the amplitude of high frequency ones [186],
Klimesch and colleagues [47, 187, 188, 189], have proposed that brain and body
oscillations are harmonically related, and form a binary hierarchy of center
frequencies (f
This article has addressed evidence supporting the idea that transduction of cardio- and respiratory-induced ICP pulsations, underlies a novel, non-synaptic mechanism of information processing by the brain. However, the idea that the heart and brain are functionally linked can be traced back, at least to Claude Bernard’s 1867 essay “Lecture on the Physiology of the Heart and Its Connections with the Brain” ([191], and see also [33, 192]) in which he described the vasculature and pneumogastric (i.e., vagal) nerve connections between the brain and heart (Fig. 9A, Ref. [191]). Today, the two-way neural links between heart, lungs, and brain (Fig. 9B) are well recognized, and underlie several important physiological phenomena (e.g., HEP, HRV and RSA). Specifically, the mechanical oscillations of the heartbeat and of breathing are synaptically transmitted to multiple brain regions where they modulate sensory, emotional, and cognitive function [33, 34, 35, 36, 38, 39, 40, 41, 42, 192]. Considering the multiple external neural inputs that exist, the question arises whether the proposed non-synaptic IRD mechanism has special functional significance apart from reinforcing the synaptic mechanisms. One idea is that the IRD is predominate in humans because it offers several advantages. Specifically, because the human brain is ~3250 times larger in volume than the mouse brain (i.e., ~1300 mL vs. ~0.4 mL), there may be limitations in using slow, energetic-costly, long axonal pathways to synchronize remote neural networks, particularly when compared to the advantages of synchronization by fast ICP-pulse transmission (e.g., ~1500 m/s). Moreover, the cardio-respiratory-pressure pumps provide, in addition to oxygenated blood, a “perpetual” supply of mechanical energy that the brain can utilize in the IRD mechanism. Indeed, the advantages of speed and lower energy costs have been used to argue for synchronization by electrical oscillations [45].
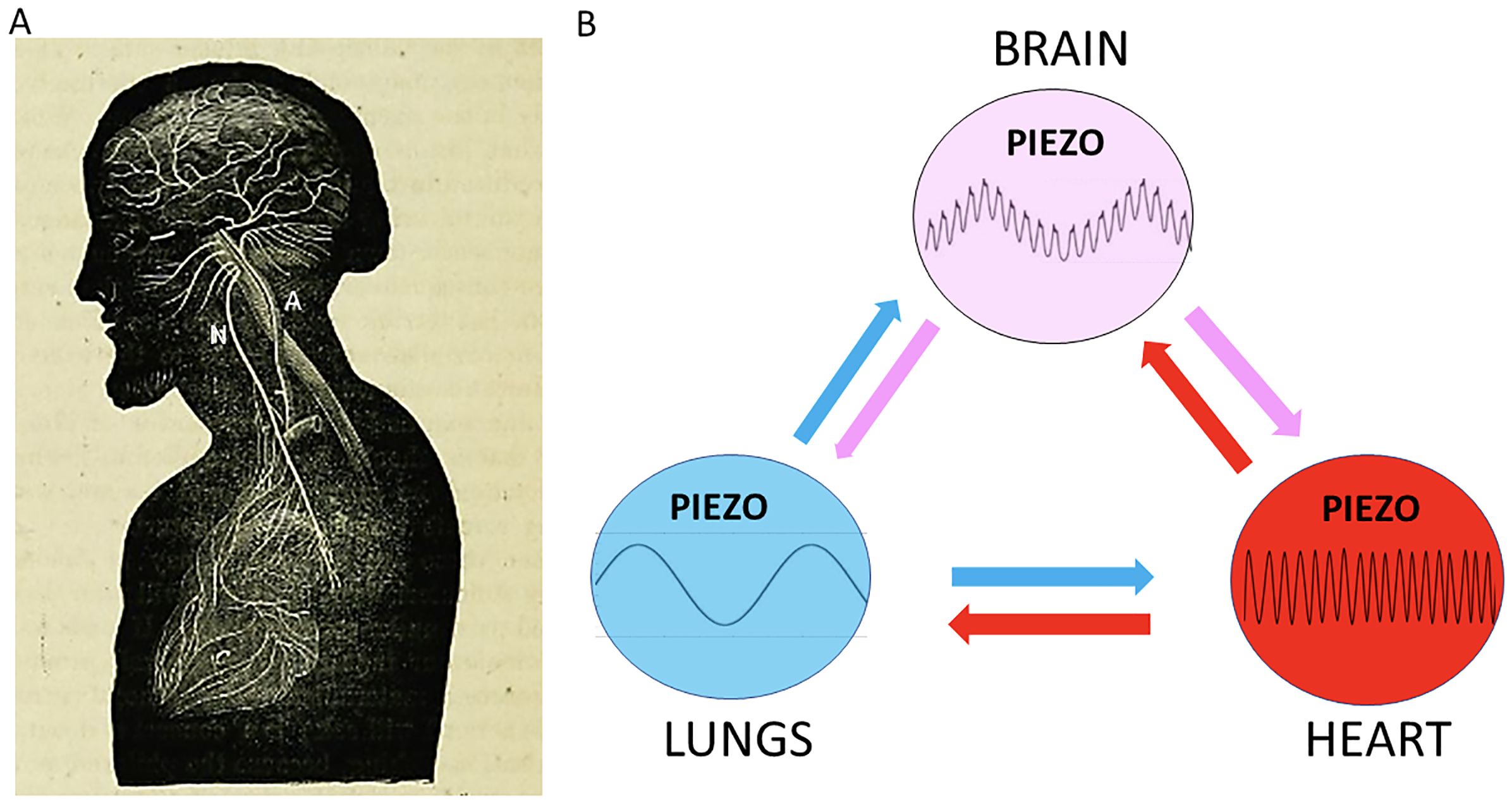
Heart-lung-brain rhythms involving extracranial and intracranial pulsatile pressure cycles transduced by Piezo channels reciprocally interact. (A) An early model of heart-brain interactions represented in a drawing showing vascular (A: carotid artery) and neural (N: vagal or pneumogastric nerve) connections between the heart and brain. Reproduced from Claude Bernard’s 1865 Lecture on the “Physiology of the Heart and Its Connections with the Brain” Delivered at the Sorbonne, the 27th March, 1865. Purse, 1867 [191]. (B) Schematic representing the reciprocal interactions between heart, lungs, and brain. The lung/breathing rhythm (blue) regulates the heart rate, by the process referred to as respiratory sinus arrhythmia. The heart rhythm (red) regulates lungs/breathing by a poorly understood process referred to as cardiorespiratory coupling in which peak systolic blood pressure initiates inspiration. The heart and brain interact in several ways, most notably through the baroreflex. In addition, the heartbeat evoked potential and heart rate variability impact the brain via afferent inputs to a wide range of brain regions. The lungs/breathing interact with the brain in several ways, most notably via nasal breathing that promotes respiration-related brain oscillations also referred to as respiratory olfactory reafferent discharge. Finally, it is proposed that breathing (~0.2 Hz) and cardiac (~1.25 Hz) rhythms generate ICP pulsatile cycles (pink) that synchronize neuron activity within remote neural networks via intrinsic resonance discharge.
IRD has also been suggested to play a more significant role in humans than in other mammals because nasal breathing is not obligatory and may be volitionally switched to mouth breathing under specific circumstances. One dramatic example is human freediving, which involves respiratory-induced behavioral changes to meet the stresses of a deep dive [130]. Freedivers typically do a relaxation breathe-up, involving several minutes of slow, diaphragmatic, and exhale-biased snorkel breathing, while floating face down on the surface of the water. This practice evokes a very specific set of neurological, physiological, and psychological outcomes that allow for the exceptional experience of diving to depths as great as 200 meters [130, 131]. The fact that the breathe-up involves snorkel breathing supports the idea that ICP pulsatility is what promotes the respiratory-related oscillations and the related brain state required for freediving [130]. Furthermore, once the dive commences, cardiac-induced ICP pulsatility may modulate in a top-down manner the reduction in heartbeat rate (~10 beats/min) along with the ongoing changes in sensory and cognitive functions [130, 193].
The future challenge regarding the IRD mechanism remains in demonstrating that it operates in vivo in the CNS as convincingly as the cardio-respiratory pulse entrainment of peripheral mechanoreceptors have provided “proof-of-concept” for the IRD in the peripheral nervous system [30, 31]. A somewhat similar challenge was recently successfully met using noninvasive optogenetics to evoke tachycardia, and to demonstrate enhanced anxiety-like behavior in risky contexts, confirming in vivo both brain and heart involvement in triggering specific emotional states [194]. However, the major challenge for the IRD mechanism is isolating its action from those of the ORD and RCD mechanisms. One obvious approach is to conduct cell-attached patch-clamp recordings from brain neurons [16] in anesthetized or awake animals [72, 195]. Currently, only single unit recordings from cat and human brain using microwire electrodes have detected correlated cardio-respiratory discharge [196, 197, 198]. However, the absence of patch-clamp evidence may reflect an issue of focus rather than evidence of absence. For example, it took ~25 years after an early whole-cell patch study of neocortical pyramidal neurons [199] to test for pressure-activated channels in cell-attached patches [16].
A different approach to identify IRD mechanism in relative isolation may come from studies of ICP pulsatility in the spinal cord, particularly in lower non-mammalian vertebrates. Specifically, ICP measurements in freely moving alligators indicated sinusoidal ICP pulsations of ~60 mmHg and ~0.5 Hz that correlated with the alligator’s undulated locomotion, and which disappeared when movement stopped [200]. These ICP pulses are ~15 times larger than the alligator’s (and human’s) cardiac-induced ICP pulsations (~4 mmHg) [200]. Those results take on added significance with the identification, initially in alligator [201] but subsequently in fish and mammal, including humans, of neurons in spinal-cord white matter that are referred to as edge cells, and which have been shown to be mechanosensitive [202, 203, 204]. Moreover, edge cells in zebra fish express Piezo2 and are proposed to act as central proprioceptors of spinal cord movement [204]. These results, and the fact that neither ORD nor RCD mechanisms should operate on spinal cord edge cells either before or during locomotion, may prove ideal in isolating Piezo2/ICP mediated IRD in the CNS.
A recent preprint [205] by Egger and colleagues reports—using a semi-intact rat nose brain preparation and a peristaltic pump to apply arterial pressure pulsations to the cerebral vasculature—that pressure pulsations induce local field potentials within the OB, consistent with fast activation of Piezo2 channels in mitral cells. In addition, in awake animals it was found that the spiking of some mitral cells is entrained to the heartbeat, also consistent with a fast baroreceptor transduction mechanism.
OPH wrote the manuscript.
Not applicable.
I thank Dr. Richard Coggeshall for critically reading the manuscript and the anonymous reviewers for their comments and suggestions.
This research received no external funding.
The author declares no conflict of interest. Owen P. Hamill is serving as one of the Editorial Board members and Guest editor of this journal. We declare that Owen P. Hamill had no involvement in the peer review of this article and has no access to information regarding its peer review. Full responsibility for the editorial process for this article was delegated to Gernot Riedel.
Publisher’s Note: IMR Press stays neutral with regard to jurisdictional claims in published maps and institutional affiliations.