- Academic Editor
Recent studies have shown that the gut microbiota regulates intestinal function and maintains intestinal homeostasis, as well as interacting with the central nervous system to affect brain function and human behavior. Microglia are the most common immune cell type in the central nervous system during homeostasis. These cells play an important role in immune surveillance by responding to infections and other pathological conditions. Microglia also play a major role in maintaining brain homeostasis in both developing and adult mice by phagocytosing cell debris and regulating the formation of neural networks. The specific signaling pathways and cytokines that control the maturation and activation of microglia are currently not fully established. However, research on germ-free (GF) mice and specific pathogen-free (SPF) mice indicate that gut microbiota have important interactions with microglia. Here, we review the latest research findings on how gut microbiota can affect the morphology, maturation, phenotype and function of microglia. We also discuss recent advances in the gut microbiota-microglia-disease axis.
Interaction between gut microbiota and the human body, especially the central nervous system, has been demonstrated in numerous studies. These includes studies of the gut-brain axis, as well as animal models of microbiota and brain diseases. Gut microbiota can have bidirectional communications with the brain, thereby affecting behaviors such as social interaction [1], depression-like behavior [2] and anxiety-like behavior [2]. Gut microbiota also show close relationships with the pathological course of several neurological inflammatory diseases. The four main ways in which gut microbiota can affect host brain activity include the vagus nerve pathway [3], the neurotransmitter pathway [4], the neuroendocrine pathway [5], and the immune pathway [6]. The mechanism by which hosts regulate their gut microbiota is thought to be via miRNA control of bacterial gene expression [7], with the miRNAs originating from the host epithelium.
Microglia arise from the embryonic yolk sac and are the most common immune cells residing in the brain parenchyma. They are involved in a wide variety of processes in the central nervous system, including defense against infection, mediating inflammation, and phagocytosis of cell debris. Recent studies have found that microglia play important roles in neurodevelopment in addition to traditional macrophage functions. These include: (1) regulation of neuronal cell programmed death [8], (2) promotion of axon formation, synapse generation, and fasciculation of axons [9, 10], and (3) stripping of excess synapses from developing neurons in order to form functional neuronal circuits [11]. Such important functions mean that microglia also play key roles in several diseases of the nervous system, including Alzheimer’s disease (AD) [12], Parkinson’s disease (PD) [13], multiple sclerosis (MS) [14] and glioma [15].
Microglia have a variety of shapes, including ramified-, amoeboid-, hypertrophic-, rod-, dystrophic-, and satellite-type [16]. The morphology of microglia is thought to be closely related to their function. Rod-shaped microglia participate in the detachment of presynaptic membrane from its corresponding postsynaptic membrane [17]. Amoeboid-shaped microglia exist mainly in the developing central nervous system and during some acute infections of the central nervous system, where they are thought to be involved in myelination and in certain inflammatory processes, respectively [18]. Compared with specific pathogen-free (SPF) mice, the microglia of germ-free (GF) mice have a ramified-type morphology, with longer protrusions and more branch and end points [19]. Moreover, they do not show a normal immune response to Lipopolysaccharide (LPS) or to viral infection. Highly branched microglia are also seen in mice models after long-term treatment with broad-spectrum antibiotics. Researchers have also found that microglia morphology in a mouse model of Alzheimer disease (AD) is affected by the microbial population in a gender-specific manner [20]. In male AD mice treated with broad-spectrum antibiotics, the microglia are clustered around amyloid plaques and show smaller cell bodies, longer tree-like branches, and more branching points. On the other hand, the microglia in female AD mice show a larger cell body, and no significant changes in the tree-like branches and branch points. The pathological process in male AD mice was also found to be attenuated after treatment with broad-spectrum antibiotics. In a mouse model that combined malnutrition with iterative exposure to fecal commensals, the microglia showed a smaller cell volume and territory, but no significant change in the number of endpoints and branch points [21]. Thus, gut microbiota has an important influence on the morphology of microglia. Although the function of microglia appears to be closely related to their morphology, the specific mechanism involved is unclear and requires further research.
Microglia originate from the yolk sac of the embryo. They enter the brain when the first neurons are generated at around embryonic day 9.5 in mice (E9.5), and self-renew in adulthood. Erny et al. [19] found that gut microbiota is an important factor in the maturation and gene expression of microglia. RNA sequencing analysis revealed that microglia RNA transcripts from GF mice are significantly different to those of SPF mice.
Some genes related to microglia activation and immune function are
down-regulated in GF mice compared to SPF mice, including Mapk8, Fcgr2
Investigators have also studied Altered Schaedler Flora mice (ASF mice, mouse model with 8 strains of bacteria) in addition to SPF mice [19]. Although the ASF mice and SPF mice were made to have the same microbial load, their microglia were still very different in terms of morphology, function and maturity. This study showed that single or several different bacterial populations do not help microglia to mature, and that maturation requires complex and diverse microbial populations. By transplanting the microbiota of SPF mice, the immature microglia phenotype of GF mice can essentially return to a normal state and full maturity can be reached in adulthood. Adult SPF mice show an immature microglia phenotype following treatment with broad-spectrum antibiotics. Therefore, the involvement of microbiota on the maturation of microglia continues from embryonic development through to adulthood. The above research findings confirm that microglia in GF mice are in an immature state, but can return to a normal state after the intestinal microbiota is restored. This suggests a close connection between the maturation state of microglia and the intestinal microbiota, although the specific mechanism requires further in-depth study.
Similar to peripheral macrophages, microglia have different states under
different stimulating factors. Researchers have simplified these into the M1 and
M2 phenotypes in order to distinguish two different polarization states [23, 24, 25].
Polarization to M1 phenotype after stimulation with IFN-
The use of M1/M2 phenotypes helps to simplify our understanding of the functional heterogeneity of microglia. However, it remains controversial whether the the M1/2 phenotype concept, originally applied to peripheral macrophages, can also be applied to microglia [32]. Furthermore, three subsets (M2a, M2b, and M2c) were observed in M2 phenotype macrophages. M2a and M2c macrophages inhibit inflammation and promote tissue repair, while M2b macrophages have both proinflammatory and anti-inflammatory functions [33, 34]. Whether these phenotypes also exist in microglia is still unknown. Nevertheless, a close relationship clearly exists between gut microbiota and the phenotype of microglia, thus providing an interesting framework to study the specific mechanism of the microbiota-gut-brain axis.
The function of microglia is clearly affected by its morphology, phenotype and
maturity, with the gut microbiota being closely linked to these influencing
factors. Most studies on the association between intestinal microbiota and
microglia function focus on the regulation of neuroinflammation by microglia.
Lactobacillus plantarum MA2 isolated from traditional Tibetan kefir grains can
improve cognitive disorder and anxiety-like behavior in a mouse model of AD, as
well as reduce the deformation of neurons and
At present, the possible mechanisms of gut microbiota and its related products
entering the central nervous system to regulate microglia mainly include direct
and indirect ways. In the direct pathway, gut microbiota transmits information to
the brain through stimulation of vagus nerve. In ischemic stroke, stimulation of
the vagus nerve has been found to modulate microglia M1/M2 type activation [40].
Vagotomy may have the effect of delaying the progression of AD pathology [41]. In
addition, metabolites of the intestinal flora including short-chain fatty acids,
secondary bile acids and TMAO have modulatory effects on neurodegenerative
diseases [42, 43, 44]. These products are characterized by low molecular weight and
high lipid solubility, so they can cross the blood-brain barrier to the central
nervous system. In the indirect way, the gut microbiota promotes the production
of pro-inflammatory cytokines such as IL-1
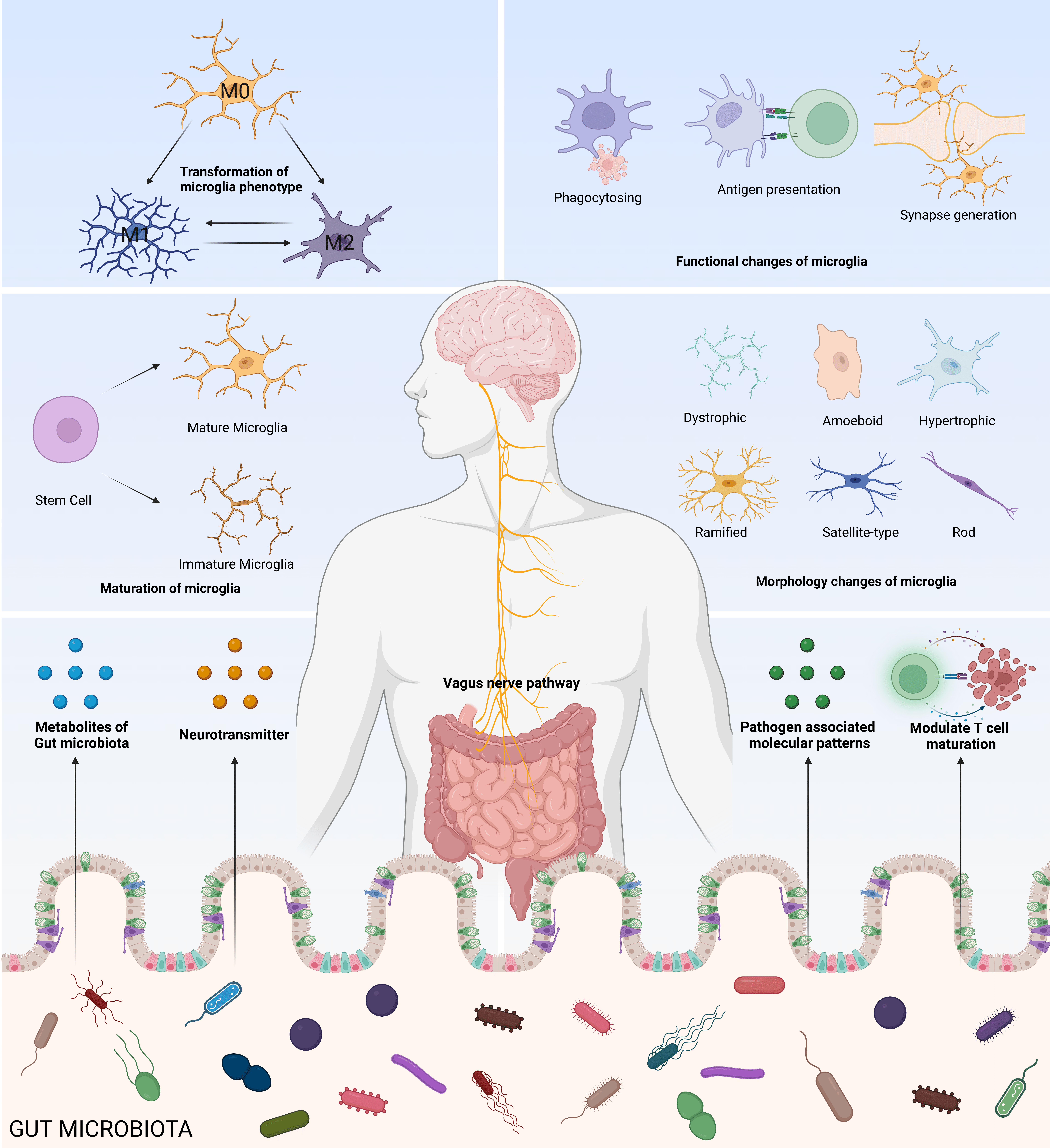
Gut microbiota-associated substances modulate maturation, morphology, function and phenotype of microglia. Direct pathway includes gut microbiota metabolites, stimulation of the vagus nerve. Indirect pathway includes secretion of neurotransmitters, effect of PAMPs on innate immunity via PRR and regulation of T cell maturation.
Microglia express a variety of innate immune-related pattern recognition
receptors, including Toll-like receptors, NOD-like receptors, and scavenger
receptors, which are essential for their recognition and response to PAMPs of gut
microbiota. The transcription products of almost all Toll-like receptors are
expressed in microglia, including TLR1-9, but not all TLRs are translated into
proteins [48]. Only TLR1,2,3,4 and 9 have been found to be expressed at the
protein level in primary rodent and human microglia cell lines [48]. TLR4
responds to bacterial LPS and promotes microglia paracrine activation [49], while
TLR2 activation promotes antagonism against Gram-positive bacteria in the CNS
[50, 51]. TLRs, upon receiving LPS stimulation, pass through their intracellular
part to promote cell signaling, induce microglia to polarize toward M1 type and
secrete proinflammatory factors such as THF-
The intestinal microbiota can anaerobically ferment undigested food in the colon into extremely diverse metabolites. The intestinal barrier, composed of intestinal epithelial cells, allows these metabolites to enter the cells and to regulate the immune system and disease [56].
The gut microbiota can ferment undigested complex carbohydrates into organic acids, gases and short-chain fatty acids (SCFAs) through a series of complex enzymatic reactions. SCFAs include acetate, propionate and butyrate. Their concentration in the intestine is determined by the composition of the intestinal microbiota and the dietary fiber content of food. SCFAs have been found to regulate the immune status in humans and the development of multiple diseases [42, 57, 58, 59].
Erny et al. [19] first reported that SCFAs produced by the gut
microbiota could influence the maturation and function of microglia in the
central nervous system. They found that dietary supplementation with SCFAs could
promote partial re-maturation of immature microglia in the brains of GF mice and
mice treated with broad-spectrum antibiotics. The expression of CSFR1, a marker
of immature microglia, was reduced after supplementation with SCFA. In a follow
up study, these authors studied the mechanism by which acetate drives microglia
maturation and functional changes [60]. Comprehensive analysis of mitochondrial
function revealed selective complex II damage in the microglia mitochondria of GF
mice. Acetate supplementation was found to restore defective mitochondrial
function in microglia by replenishing tricarboxylic acid cycle intermediates.
Intestinal-derived acetate labeled with C
Several studies have shown that SCFAs can cross the blood-brain barrier [63, 64], suggesting they may act directly on microglia to affect their functions. SCFAs can be bound by specific receptors, such as Free Fatty Acid Receptor 2 and 3 (FFAR2, FFAR3) [65]. Although FFAR2 is not expressed in any adult brain cells including microglia and endothelial cells, FFAR2 gene-deficient mice have similar microglia to GF mice [19, 66]. This suggests that SCFAs can also bind to FFAR2 expressed by peripheral myeloid cells or lymphocytes (e.g., spleen or intestinal macrophages), thus indirectly sending signals to the central nervous system and thereby regulating the maturation and function of microglia. However, there is currently no evidence showing that brain cells express FFAR2 during early embryonic development. More research is therefore needed to understand the mechanism by which SCFAs affect microglia.
AD is the most common neurodegenerative disease and causes dementia in millions
of people worldwide. Cerebral amyloidosis and abnormal protein deposition are
typical pathological features of AD. Microglia in the brain proliferate, activate
and concentrate around these amyloid plaques and protein depositions. Genetic
studies have shown that most AD risk genes are highly and selectively expressed
in microglia, including TREM2, CD33 and APOE [67]. In-depth studies of the
microbe-gut-brain axis have led to the hypothesis that gut microbes influence the
pathological changes observed in AD by affecting microglia. Harach et
al. [68] found significantly less amyloidosis in the central nervous system of
APPPS1 transgenic mice (AD model containing human transgenes for both
APP bearing the Swedish mutation and PSEN1 containing an
L166P mutation, both under the control of the Thy1) under GF
conditions compared to SPF mice. However, the level of amyloidosis became similar
after the transplantation of intestinal microbes from specific pathogen free
APPPS1 mice. The above studies suggest that the presence or absence of intestinal
flora has an important impact on the pathology of AD. Changes in the composition
of gut microbiota were also found in the 5
PD is the second most common neurodegenerative disease after AD and is
characterized by the impairment of movement and cognitive decline. PD is caused
by the loss of dopaminergic neurons in the substantia nigra compact area, and
accumulation of
MS is a chronic neurodegenerative disease of the central nervous system mediated
by T cells. Immune cells from the central nervous system and from the peripheral
immune system are involved in the pathological process of MS. The important role
of gut microbiota in this process was demonstrated by Berer et al. [75].
These workers used a self-developed mouse model of MS to show that intestinal
microbiota and myelin autoantigens jointly induced an autoimmune demyelination
response. In follow-up work they found that Akkermansia may cause
aggravation of MS symptoms. Using Experimental Autoimmune Encephalomyelitis (EAE)
mice model (a model widely used to simulate MS), Haghika et al. [76]
showed that long-chain fatty acids can sustainably reduce the concentration of
SCFAs in the intestine, thereby increasing Th1 and Th17 cells in the small
intestine and aggravating the disease symptoms. Meanwhile, in the EAE mouse
model, treatment with a mixture of Clostridium strains reduced microglia
activation rate and improved axonal damage. In addition, Clostridium treated mice
were found to have elevated plasma butyrate levels, but treatment with butyrate
alone did not produce a therapeutic effect on EAE mouse model [77]. Further,
transplantation of fecal from healthy mice into EAE mice modulates the gut
microbiota of EAE mouse model, improves the severity of EAE by alleviating blood-brain barrier (BBB)
leakage and decreasing microglia activation [78]. It is not yet known how the gut
microbiota regulates immune cells in the peripheral and central nervous system
during the pathological process of MS, although antibiotic treatment of
lecithin-induced MS mouse models was shown to alter the central nervous system.
Inflammatory conditions such as the functional impairment of myelin fragments and
the differentiation of oligodendrocyte progenitor cells were also shown to be
affected by the activation of microglia. Recent studies have found that the Aryl
Hydrocarbon Receptor (AHR) ligand derived from gut microbiota not only controls
astrocytes but also binds to microglia that express AHR. This activates the
microglia and increases their secretion of TGF-
ASD is a severe neurodevelopmental disorder characterized by age inappropriate, impaired social communication, and the presence of stereotypic behavior. During neurodevelopment, microglia are involved in regulating the number and strategic positioning of neurons and shaping neuronal connectivity [79, 80], and also support gliogenesis and myelination [79, 81]. Due to their important functions, microglia are thought to play an important role in ASD. In a study of pathological samples, Vargas et al. [82] found increased microglia activation throughout the cerebrum and cerebellar cortex of ASD patients, as evidenced by increased MHCII expression. In another study, it was also found that microglia density was increased in the brain and cerebellar cortex of patients with ASD and exhibited enlarged cell bodies, synaptic constriction and thickening [83, 84]. Also, it was found that overproduction of proteins, such as overexpression of the transcription initiation factor EIF4e, in microglia alone was sufficient to cause mice to exhibit impaired synapse formation and ASD-like behavior [85]. Several studies have found alterations in the composition and abundance of the intestinal flora of children with ASD, with increased proportions of Clostridium, Suterella, Ruminococcus, Lactobacillus, and decreased proportions of Bifidobacterium, Akkermansia, Blautia, and Prevotella [86, 87, 88, 89, 90, 91]. Transplantation of fecal microbiota from the neurotypical control donors group into ASD patients significantly improved irritability, communication skills and sociability [92, 93]. Propionate in SCFAs increases inflammatory cytokine production and ASD-like behavior in mice by increasing the activation of microglia [94, 95]. While butyrate was found to promote the transcription of genes related to [96] neuronal inhibitor pathways, thereby improving social behavior in BTBR mouse strains, an idiopathic ASD model. There is a significant relationship between microglia function, gut microbiota and ASD, which may provide us with new therapeutic targets, but the exact mechanisms remain to be fully established.
Depression is a major mental disorder, estimated to reach approximately 4.4% of
the world population [97]. The main symptoms of Major Depressive Disorder include
depressed mood, anhedonia, irritability, difficulty concentrating, changes in
appetite and sleep, and others [98]. The main hypothetical theories about MDD
include monoamine neurotransmitter depletion hypothesis, neuroplasticity
hypothesis, hypothalamus–pituitary–adrenal [99]. Several studies have shown
that microglia are closely related to the three hypotheses mentioned above [100, 101]. Microglia play an important role in neuroinflammatory diseases because of
their functions such as activation of inflammation, synaptic refinement, synaptic
pruning, and neuronal connectivity [102]. Thus, MDD is also considered to be a
microglia-related disease. Probiotic targeted regulation of gut microbiota has
been found to be a new strategy for improving MDD. Lactobacillus reuteri NK33 and Bifidobacterium adolescentis NK98
were found to ameliorate restraint-induced stress anxiety/depression in mice
[103]. A study by Zhang et al. [104] showed that administration of an
anti-mouse IL-6 receptor antibody (MR16-1) led to antidepressant effects in a
model of socially defeat stress and an improvement in
Firmicutes/Bacteroidetes ratio in susceptible mice, suggesting
that IL-6 blockade induces antidepressant effects by normalizing the composition
of the altered gut microbiota. The kynurenine pathway (KP) plays an important
role in the development of psychiatric disorders, including schizophrenia [105]
and MDD [106]. Gut microbiota metabolites such as short-chain fatty acids can
promote 5-hydroxytryptamine production via tryptophan, thereby modulating this
pathway and preventing the conversion of tryptophan to kynurenine [107]. A small fraction
of tryptophan is metabolized to 5-hydroxytryptamine through
indoleamine-2,3-dioxygenase (IDO) enzymes, which are contained in all tissues,
while another fraction enters the kynurenine pathway [108]. During intestinal
inflammation, stimulation of the inflammatory cytokines, such as interferon
Gliomas are the most common and lethal tumor of the central nervous system in adults. About 50% of glioma patients have the most aggressive phenotype. The five-year survival rate of these patients is just 3.3%, with a median survival time of only 14.6 months [114]. Like other solid tumors, gliomas have a complex immune microenvironment that has important effects on tumor proliferation, invasion and metastasis [15]. Microglia are believed to play a role in the pathogenesis of gliomas by promoting the migration and proliferation of glial cells. The relative abundance of Akkermansia and Bacteroides increased after tumor growth in the syngeneic mouse model of glioma (GL261), but no obvious difference was found in 6 glioma patients [115]. Moreover, the concentrations of fecal microbiota metabolites such as SCFAs, 5-hydroxyindole acetic acid and norepinephrine were reduced. In another study using the GL261 mouse model, treatment with broad-spectrum antibiotics promoted the growth of mouse gliomas and altered the subpopulation and function of NK cells in the brain, bone marrow, and spleen [116]. Although they did not find a significant change in the density of microglia, these authors found that antibiotic treatment increased the expression of Arg1, P2ry12 and INOS in the brain. Some researchers have speculated that the observed phenomenon may be due to the lack of signals from Prevotellaceae, Rikenellacaea and Helicobacteraceae, or to the up-regulation of signals from Burkholderiales. In summary, the research to date on regulation of microglia by intestinal microbes and the possible effects on glioma development is still preliminary. Further research is needed to clarify these relationships, including identification of the specific mechanisms or signaling pathways.
Multiple experiments have shown that the intestinal microbiota regulates peripheral immune cells, thereby affecting malignant tumors of the digestive system. For example, it was recently reported that the intestinal microbiota of colorectal cancer patients can stimulate cathepsin K secretion, mediate the polarization of TLR4 receptor-dependent M2 microglia, and promote tumor metastasis [117]. Microglia are considered to be macrophages in the central nervous system. Intestinal microbiota could in theory also change the polarization state of microglia, thereby affecting the microenvironment of central nervous system tumors and of brain metastases.
Recent studies have shown that the intestinal microbiota can affect not only the occurrence, invasion and metastasis of tumors, but may also impact the efficacy of tumor treatment. The efficacy of cyclophosphamide, a traditional and widely used cancer drug, in a mouse tumor model has been shown to depend on the intestinal bacteria. Mice with solid tumors showed a reduced Th17 response under sterile conditions or with the use of broad-spectrum antibiotics. The tumors were also resistant to cyclophosphamide. Specific strains (e.g., B. fragilis) in GF mice may overcome tumor resistance to CTLA-4 immunotherapy [118]. In light of the above findings it is likely that microglia, as the only resident immune cell in the brain, are also regulated by the intestinal microbiota. Therefore, microglia occupy an important position in the development of brain tumors (primary or metastatic) and in treatment response. The intestinal microbiota can affect brain tumors by regulating microglia, suggesting this axis may provide a novel therapeutic target as well as new directions for future studies of brain diseases.
Perhaps five years ago, it would have been difficult to imagine such a broad and tight connection between the intestinal microbiota and microglia, which could serve as an axis through a wide variety of disease pathologies and as potential therapeutic targets. Because of the importance of these connections, we need to go back to the roots of these connections and dig deeper into the underlying mechanisms. First, the pathways through which the gut microbiota affect microglia are diverse and can be functionally oriented to investigate the specific mechanisms of each regulatory pathway and possible synergistic effects in disease models. Second, the regulation of metabolism on the polarization state of microglia can be studied from a metabolomics perspective, similar to the regulation of peripheral macrophage metabolism. Third, the existence of the concept of M1/M2 type polarization of microglia is still controversial and can be studied from the perspective of individual cell genomics, proteomics and metabolomics to expand the understanding of the microglia phenotype.
XD and FT designed the study. LL, HL, YB, PD, LP and ZL conducted data collection. LL wrote the first draft of the manuscript. All authors contributed to the manuscript revision and read and approved the final version. All authors have participated sufficiently in the work to take public responsibility for appropriate portions of the content and agreed to be accountable for all aspects of the work in ensuring that questions related to its accuracy or integrity.
Not applicable.
Not applicable.
This work was supported by the National Natural Science Foundation of China (81573090, 81172595, 81703165).
The authors declare no conflict of interest.
Publisher’s Note: IMR Press stays neutral with regard to jurisdictional claims in published maps and institutional affiliations.