- Academic Editor
†These authors contributed equally.
Introduction: Painful diabetic neuropathy (PDN) is an
intractable chronic pain condition affecting a growing number of adults in China.
Spinal cord stimulation (SCS) has been employed in the treatment of PDN for
several decades. However, the efficacy and underlying mechanisms of SCS are still
inconclusive. Methods: In this study, we adopted an implantable pulse
generator to deliver electrical stimulation (50 Hz, 200 us pulse width, 12
hours/day in 5 weeks) via a quadripolar electrode in the lumbar epidural space to
treat pain hypersensitivity in the rat model of PDN. Electronic von Frey and
Hargreaves tests were used to measure the responses to mechanical and heat
stimuli, respectively. Quantitative PCR, western blotting, and enzyme-linked
immunosorbent assay (ELISA) were adopted to explore the changes in
neuroinflammation after SCS. Results: SCS alleviated mechanical
allodynia and heat hyperalgesia over a period of 3 weeks in diabetic rats. SCS
completely suppressed neuropathy-induced Tlr4 and NF
The prevalence of diabetes is increasing in China. It was estimated that total diabetes and newly diagnosed diabetes account for 12.8% (12.0–13.6%) and 6.8% (6.1–7.0%) of adults in China [1]. Meanwhile, 50% of these patients may develop peripheral neuropathy in their lifetime [2] and up to 50% of patients with diabetic neuropathy may suffer from neuropathic pain syndrome [3]. Hence the painful diabetic neuropathy (PDN) gains growing attention [4]. Unfortunately, the pharmacological treatment for PDN is still not satisfactory in clinical practice.
Diabetic neuropathy is characterized by the loss of small fibers in the skin and is generally regarded as peripheral neuropathy. Pathological alterations in the dorsal root ganglion (DRG) are one of the most discernible etiologies of painful neuropathy. For instance, it was reported that 1/3 of c-fibers (nociceptors) exerted hyperexcitability in response to mechanical stimuli, resulting in an up to a 3-fold increase in firing frequency [5]. Cxcl12 appeared to cause the hyper-responsiveness of c-fibers by activating Cxcr4 [6]. Recently, the investigation of the plasma of patients with diabetes revealed that patients with PDN had significantly higher level of methylglyoxal than patients without pain. Moreover, methylglyoxal dramatically increased the excitability and firing rate of nociceptors via modulating Nav1.8 and Nav1.7 [7]. These studies suggested that the alterations in peripheral inflammation or metabolomics might evoke pain hypersensitivity via targeting at DRG. In addition, the pathological changes in the spinal cord are also discerned, given that pain hypersensitivity is an evident clinical manifestation. For instance, long-term hyperglycaemia was reported to cause microvascular complications in the spinal cord and associated with pain-like responses in the preclinical models of PDN [8]. Meanwhile, the increased number of synapses [9], oxidative stress related damage [10], infiltration of blood-borne macrophages [11] and re-activation of microglia [12] were identified as contributing factors of PDN. In this connection, spinal cord is recognized as a potential target for PDN intervention.
Since few decades ago, electrical spinal cord stimulation (SCS) has been applied as an approach for PDN therapy [13]. In this pilot study, 10 patients with treatment-refractory PDN received SCS treatment and achieved significant pain relief for up to 6 months. Numerous clinical studies further presented a promising efficacy of SCS on PDN [14, 15]. A long-term follow-up study had reported that SCS led to 50% pain reduction in 55% participants for as long as 5 years [16]. However, according to the recommendations of the international association for the study of pain Neuropathic Pain Special Interest Group (NeuPSIG), the effects of SCS on PDN is still inconclusive due to relative low quality of clinical trials [17]. The clinical guideline for the SCS treatment of PDN, such as the recommended electrical frequency, pulse width, duration of stimulation, electrode configuration, was not developed. Further studies that aim to modify the stimulation protocols could improve the therapeutic efficacies and reduce the safety concerns in the clinical practice. In this regard, preclinical studies adopting biochemistry based methodologies to objectively evaluate the changes in pain associated molecules serve as valuable approaches to fine tune SCS protocols.
In this study, the efficacy of a SCS protocol in the pain-like hypersensitivity was investigated on the rat models of PDN. The changes in pain-associated molecules, i.e., inflammatory cytokines and their upstream modulators, were also assessed after SCS.
Male Sprague Dawley (SD) rats, weighting 225 g was used in all presented experiments. Rats were kept in the animal room at a temperature of 24 °C, relative humidity of 60% and 12:12-hour light: dark cycle. All the procedures were approved by the animal ethical committee of Jingzhou Hospital Affiliated to Yangtze University (approval number: JZYY-2020142).
The diabetic neuropathy was induced via intraperitoneal (i.p.) injection of streptozotocin (STZ) as described by previous report [18]. STZ was obtained from TOCRIS (No. 1621, Bio-Techne China, Shanghai, China) and dissolved with sodium citrate buffer (10 mM, pH5.5) to make a 50 mg/mL working solution. For model development, rats were fasted for 6 hours and then injected with a single dose of STZ (45 mg/kg) using a 1 mL syringe. For sham group, rats were administrated with sodium citrate buffer. After injection, all rats were given 10% sucrose water in a period of 48 hours to prevent hypoglycaemics.
AccuChek Performa (Roche Diagnostics, Mannheim, Germany) was adopted to measure non-fasting blood glucose of rats. The blood was taken by puncturing the tip of tail with a hypodermic needle and loaded onto the test strip for measurement. The test was conducted before STZ injection and every week from day 21 to day 49 after injection. The body weight was then recorded after blood test.
The allodynia-like response of rats were measured with an electronic von Frey aesthesiometer (No. 76-0976, Harvard Apparatus, Holliston, MA, USA). The rats were placed in the light chamber on the wire mesh stand. Rats were allowed to acclimate for 15 minutes before test. For measurement, the researcher stabbed the central plantar of rats with the supplied tip and lifted until a pain-like response (i.e., withdrawal, and linking) was observed. The withdrawal threshold of mechanical stimulation was shown in the von Frey meter and recorded. For each time point, all the rats were tested for 5 times in a 5 minutes intervals. The researchers were blinded to the grouping information.
The IITC plantar analgesia meter (390G, IITC life science, Woodland Hills, CA, USA) was applied to measure the changes in heat-evoked response before and after STZ injection. Rats were place in the animal enclosure and allowed to acclimate for 15 minutes before test. A beam of light, which may deliver noxious heat, was aimed at the central plantar of rats until a pain-like response was observed (withdrawal or linking), and the paw withdrawal latency was recorded. For each time point, all the rats were tested for 5 times in a 5 minutes intervals. The researchers were blinded to the grouping information.
The quadripolar SCS electrode and Interstim-II Implantable Pulse Generator (IPG) was obtained from Medtronic (Model 8840, Minneapolis, Minnesota, MN, USA) and implanted on rats from all groups, i.e., control group (without STZ injection), the STZ+sham therapy group (n = 6) and STZ+SCS therapy group (n = 6), at week 3 after i.p. injection. Rats were deeply anaesthetized with isoflurane. A partial laminectomy was performed at the vertebrate level of L1. The electrode was then inserted towards T13 vertebrate level to cover the lumbar spinal cord. The lead of electrode was fixed to the muscles with sutures to secure its position. A IPG was then placed subcutaneously near L1 vertebrate and connected to the lead. After implantation, the muscles and the skins were closed separately. To perform SCS, N’Vision clinician programmer (model 8840, Medtronic, Minneapolis, MN, USA) was adopted to control the electrical delivery by the IPG. First of all, motor threshold was determined by gradually increasing the current amplitude (4 Hz at 200 us pulse width) from 0, until reflection was observed in the hind limb or trunk. For SCS, current amplitude at 80% motor threshold, i.e., 50 Hz at 200 us pulse width was continuously delivered for 12 hours daily, from week 4 after STZ injection, to the end of experiments. For control group and sham therapy group, no currents were delivered.
The relative expression level of Tlr4 and NF-
The protein level of Tlr4, phosphorylated and NF-
The protein level of proinflammatory cytokines were measured by ELISA. All the
ELISA kits, including rat Il1
For analysis of three groups across time, two-way analysis of variance (ANOVA) followed by multiple comparisons were conducted. For analysis of four groups at a single time point, one-way ANOVA with multiple comparisons were employed. A p-value less than 0.05 was considered statistical significance.
Rats (n = 12) were assessed for the baseline of paw withdrawal threshold (PWT) and paw withdrawal latency (PWL) by electronic von Frey test and Hargreaves heat hyperalgesia test, respectively. Rats were then randomly assigned to control (vehicle treated) or STZ treated groups according to the baseline. As expected, the blood glucose levels were dramatically elevated in STZ group, compared with the control group. The hyperglycemia was observed at week 3 after STZ injection and lasted for at least for 5 weeks (Fig. 1A). Meanwhile, the body weight was gradually gained in the control group, whereas it was not in the STZ group (Fig. 1B). These results indicated the development of type I diabetes in rats after STZ injection. Furthermore, STZ rats have significantly lower PWT and PWL than control rats, from week 4 to week 7 after model development (Fig. 1C,D). Taken together, we successfully developed the rat model of painful diabetic neuropathy.
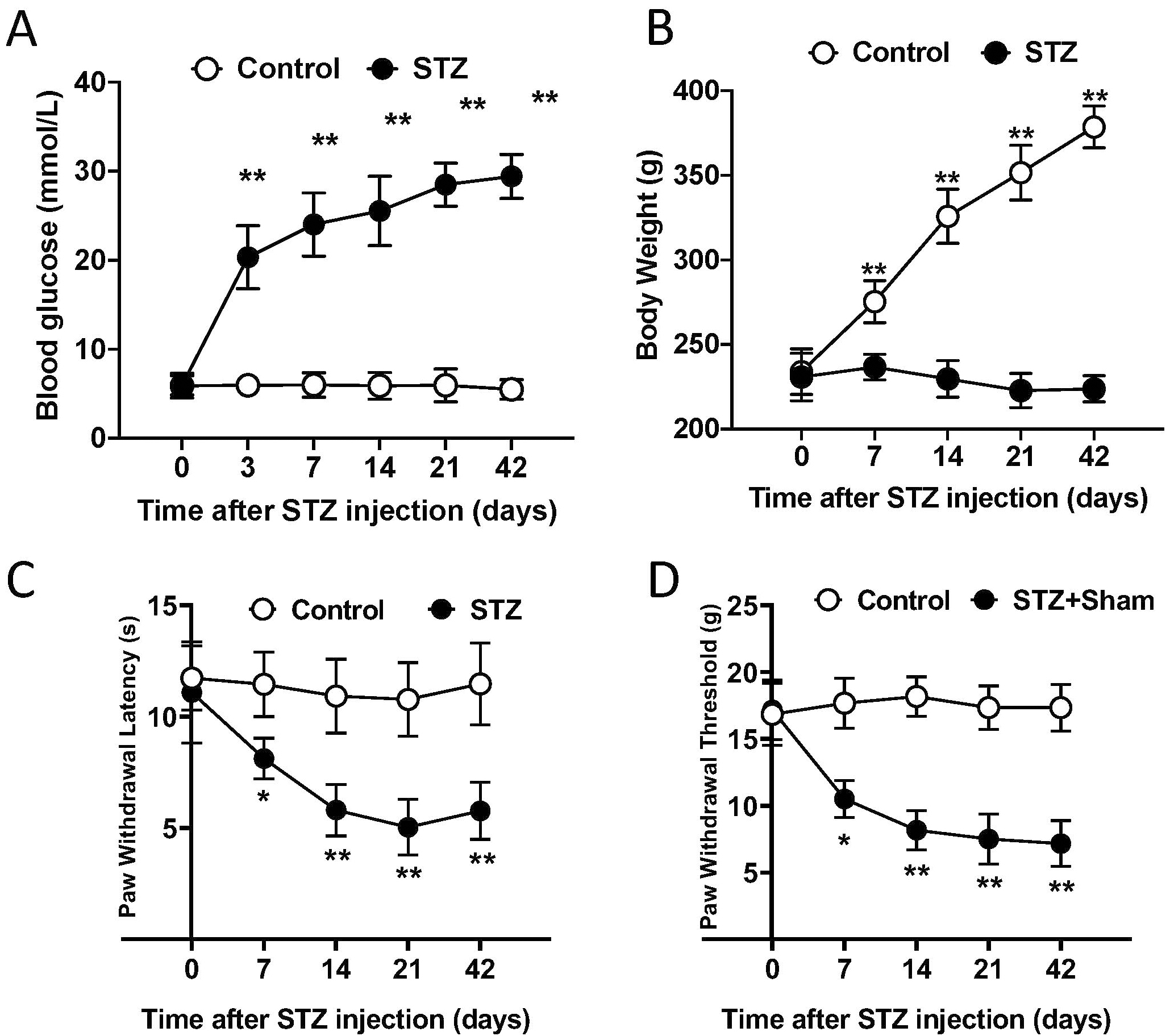
Single injection of STZ developed painful diabetic neuropathy.
(A) Blood glucose test demonstrated STZ injected rats had significantly higher
glucose levels than control rats from day 21 to day 49 after injection. (B) STZ
injection interfered with gain of body weight. (C) STZ treatment induced heat
hyperalgesia on rats from 4 weeks after injection. (D) STZ treatment induced
mechanical allodynia on rats from 4 weeks after injection. A and B, Student’s
t test. C and D, Two-way ANOVA with post hoc test. *, p
We then explored if SCS could improve diabetic neuropathy-induced mechanical allodynia and heat hyperalgesia. Rats (n = 18) were assessed for baseline of pain-like response and randomly assigned to three groups, i.e., control group, STZ+sham therapy group and STZ+SCS therapy group. The electrode and IPG implantation were conducted on rats from all groups at day 21 after model development. Then SCS (12 hours daily, 50 Hz, 200 us pulse width) or sham therapy began from day 21 to the end of experiments. As demonstrated in the Fig. 2A,B, SCS did not improve hyperglycemia and increase body weight among STZ treated rats. There was no significant difference in pain hypersensitivity between sham and SCS groups at post-injection day 21 and day 28. However, the therapeutic efficacy of SCS was evident at day 35, day 42 and day 49, as indicated by a significant increase of both PWL and PWT in SCS group, compared with sham group (Fig. 2C,D). These results suggested that SCS was an efficient approach for relieving diabetic neuropathy-induced pain.
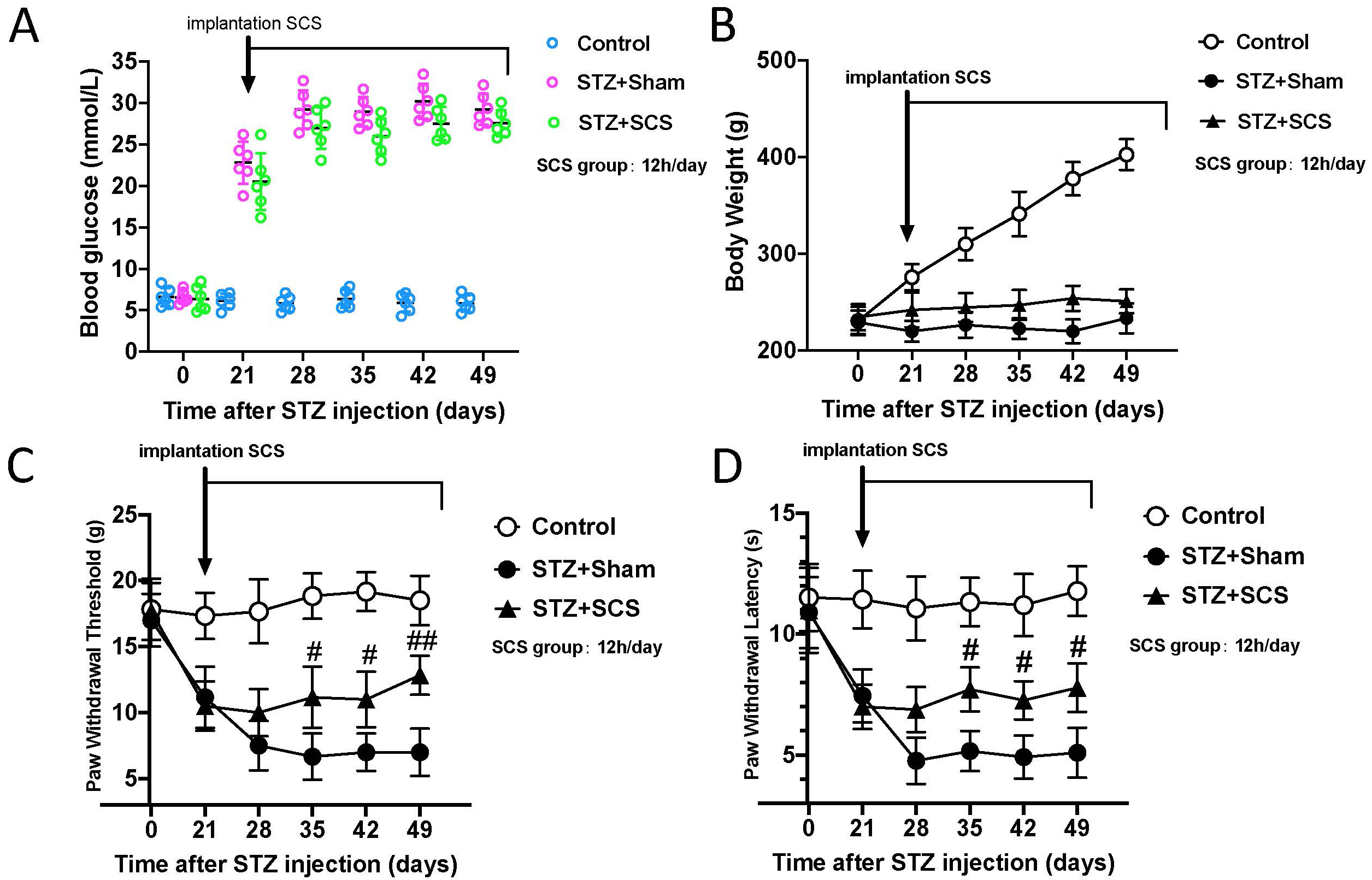
SCS alleviated pain hypersensitivity without affecting
hyperglycemia. (A) Blood glucose levels were not affected by SCS. (B) SCS did
not restore body weight gain. (C–D) SCS alleviated mechanical allodynia and heat
hyperalgesia after 2 weeks and lasted for at least 3 weeks. Two-way ANOVA with
post hoc test. #, p
Neuroinflammation has been closely associated to the development of central
sensitization, which contributes to allodynia and hyperalgesia in various model
of neuropathic pain [18, 19]. Therefore, the effects of SCS on neuroinflammation
were investigated in this study. qPCR was performed to assess the changes of Tlr4
and NF-
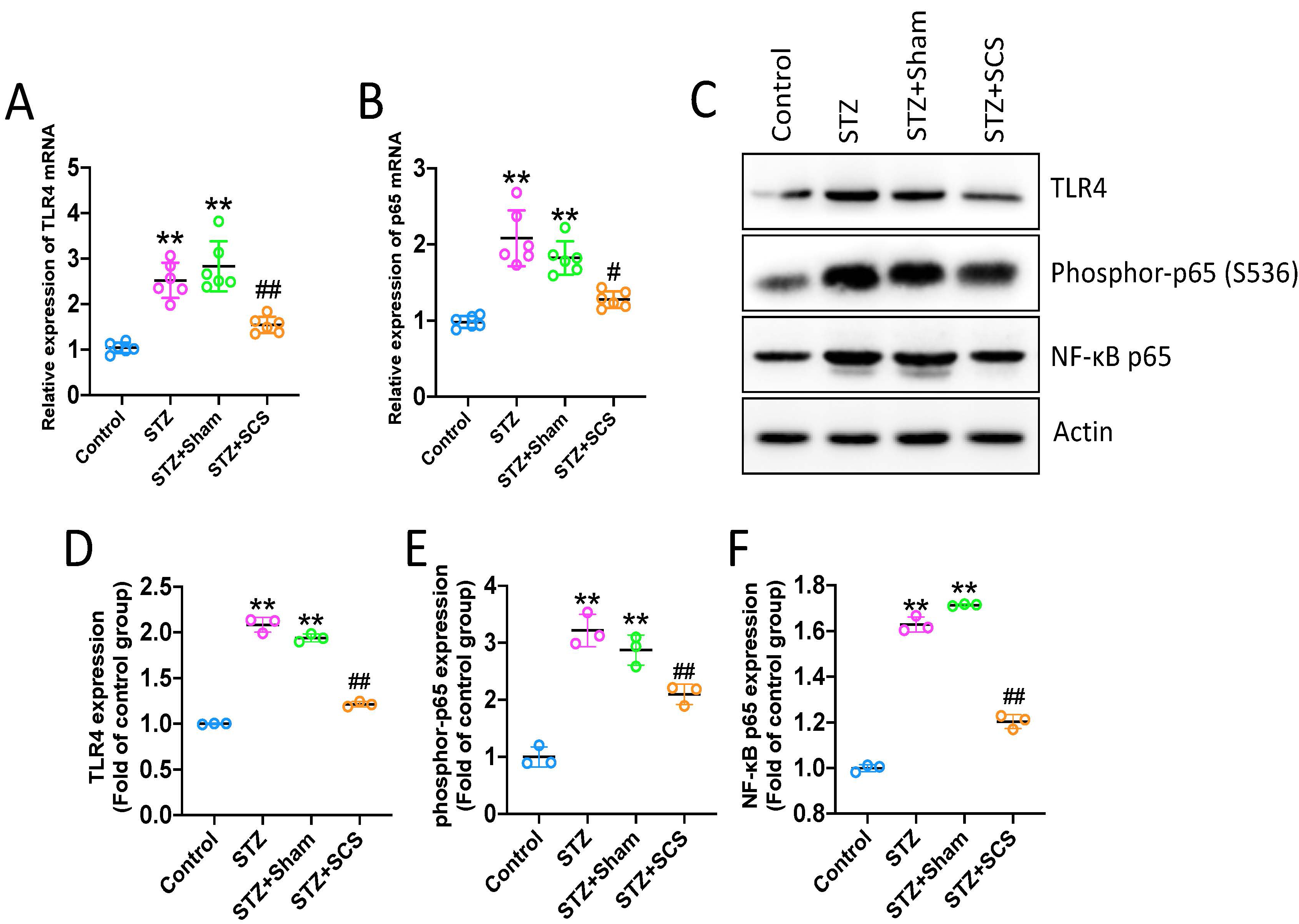
SCS suppressed Tlr4/NF-
Next, the pro-inflammatory targets of Tlr4/NF-
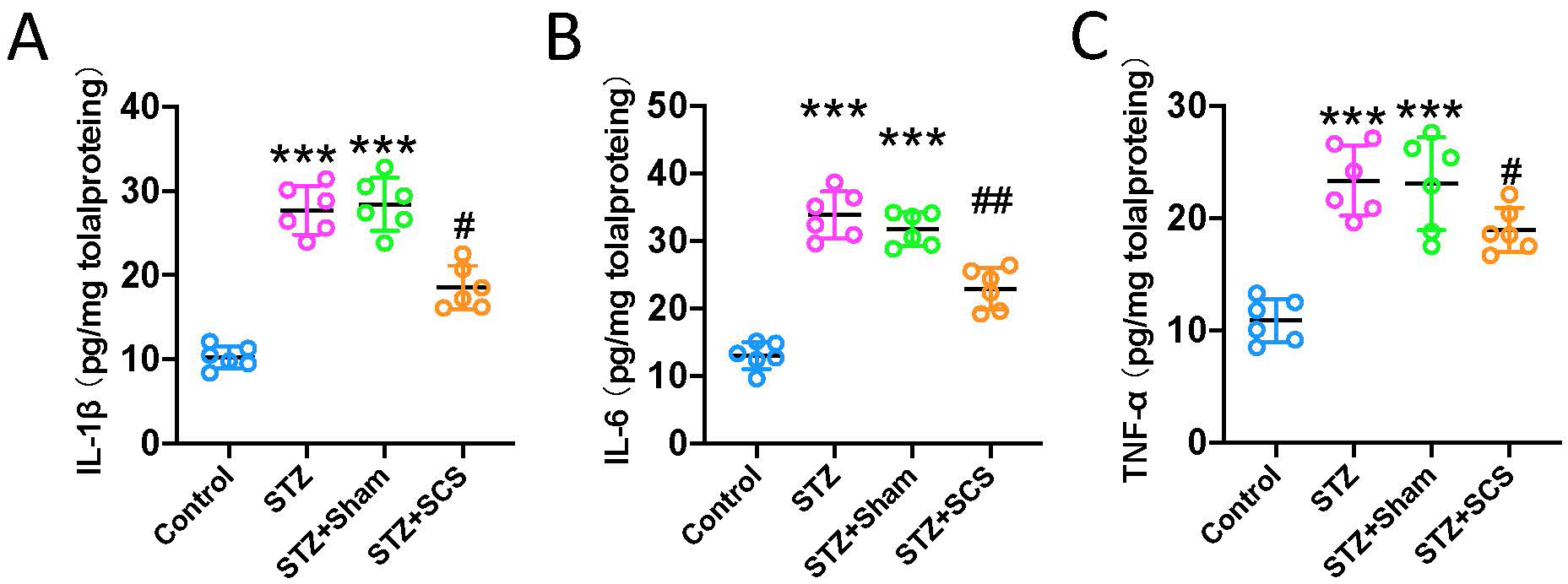
SCS reduced pro-inflammatory cytokines in spinal cord dorsal
horn. (A–C) ELISA demonstrated that diabetic rats had significantly higher
levels of Il1
In the present study, the therapeutic efficacy and potential mechanism of SCS in
painful diabetic neuropathy was investigated. We found that daily SCS for 5 weeks
successfully reduced pain hypersensitivity caused by diabetic neuropathy. The
therapeutic action was accompanied by the decline of Tlr4 and NF-
Recently, the pain hypersensitivity associated pathological changes in spinal
cord had gained more and more attentions, particularly the dysregulated
inflammatory responses. Microglia are the glia sensing injury or dysregulated
activation in central nervous system. In the model of STZ induced neuropathic
pain, microglia are rapidly aroused and the re-activation status could last for
as long as 6 months [20]. Re-activated microglia subsequently modulate
neuroinflammation by secreting a series of cytokines, such as Il1
The potential connection between neuroinflammation suppression and SCS might be useful for clinical trial design and further, for patient selection in the future clinical practice. By use of simultaneous positron emission tomography/magnetic resonance (PET/MR) imaging technique and the radiolabeled ligand of translocator protein (translocator protein, TSPO, a glia cell related inflammatory marker), spinal cord and nerve roots related neuroinflammation can be evaluated and associated with chronic pain conditions [31]. Clinical scientists are encouraged to use this technique and set up clinical trials testing if SCS analgesia is associated with the attenuated neuroinflammation in patients. Meanwhile, it is also worth investigating if patients with higher degree of neuroinflammation are more likely to be sensitive to SCS treatment. On this basis, we may recruit PDN patients having significant neuroinflammation to the priority group for SCS treatment in the clinical practice. On the other hand, after consolidating the relationship of SCS analgesia and neuroinflammation in humans, it may reinforce researchers’ confidence to seek novel approaches to targeting reactivated glia cells or neuroinflammation for PDN treatment.
There are some limitations in the current reports. While SCS exerted considerable analgesic-like actions within the duration of electrical stimulation, we did not measure the post-treatment pain thresholds after 5 weeks. It is likely that intervention on neuroinflammation may prevent the buildup of long-term central sensitization [19, 32]. Meanwhile, several independent studies had reported the efficacies of SCS to neuropathic pain by adopting differential frequencies [33, 34, 35]. In this study, only one protocol, i.e., 50 Hz was employed science a randomized controlled trial has reported that high-frequency 10 kHz SCS improves the quality of life in patients with PDN as well [36]. Therefore, further study focusing on the comparison of therapeutic efficacies between different protocols (for instance, 50 Hz vs 10 kHz) might provide pre-clinical evidence for the improved design of clinical trials. Finally, although neuroinflammation suppression has been delineated as potential mechanism of SCS, we did not pinpoint the source of cytokines (e.g., microglia and astrocytes). Previous studies suggested that microglia appeared to play critical roles only in the initiation of neuropathic pain, whereas astrocytes were activated and contributed to the maintenance of neuropathic pain [37, 38]. A precise cell type localization therefore may advise the time window of SCS delivery and refine the design of clinical trials. We will explore these open questions in the future studies.
SCS may alleviate diabetic neuropathy-induced pain hypersensitivity via attenuating neuroinflammation in the spinal cord dorsal horn.
All data generated or analyzed during this study are included in this published article.
WN, NW, YW—extraction and drafting of the manuscript; WN, JL, QX, NW—analysis of data, manuscript revision; NW, and YW—design and revision, statistical analysis. All authors contributed to editorial changes in the manuscript. All authors read and approved the final manuscript. All authors have participated sufficiently in the work and agreed to be accountable for all aspects of the work.
All the procedures were approved by the animal ethical committee of Jingzhou Hospital Affiliated to Yangtze University (approval number: JZYY-2020142).
Not applicable.
This work was supported by grants from the Natural Science Foundation of Hubei Province (No. 2018CKB920).
The authors declare no conflict of interest.
Publisher’s Note: IMR Press stays neutral with regard to jurisdictional claims in published maps and institutional affiliations.