- Academic Editor
Background: Psychosocial stress factors, such as threat and defeat, are major risk factors for the development of depression. The precise mechanisms underlying stress-induced depression are not clearly understood because the stress response in the brain varies in a stress-frequency-dependent manner. In the current research milieu on the pathogenesis of depression, the focus is on depression-like behavioral phenotype, hypothalamic-pituitary-adrenal (HPA) axis, and hippocampal neurogenesis. However, most studies have evaluated the symptomatic features of depression at certain time points after exposure to psychosocial stress. Here, we examined the frequency-dependent effects of psychosocial stress on depression-related features in rats. Methods: In the present study, different frequencies (one, two, three, or four times) of psychosocial stress were applied to 19 male Sprague-Dawley rats using a resident/intruder paradigm. Subsequently, the rats were subjected to a stress reactivity test to evaluate HPA axis activity, following which assessments of immobility behavior in the forced swimming test (FST) and adult neurogenesis were conducted. Results: One-time stressed rats showed a decrease in immobility behavior in the FST and the amount of doublecortin (DCX)-positive cells. Two-time stress caused hypoactivity of the HPA axis. In contrast, immobility behavior and HPA axis activity were increased after four-time stress exposure, but the number of DCX-positive cells was decreased. Conclusions: Our findings suggest that psychosocial stress produces a biphasic effect on the symptoms of depression in a stress-frequency-dependent manner, which could provide insights to facilitate further pathogenesis research on depression.
Depression is one of the most prevalent psychiatric disorders, with a severe burden of disability worldwide [1, 2]. There is a large body of evidence indicating that stressors contribute to the development of depression [3, 4]. However, despite intensive research on the relationship between stress and depression, the exact molecular mechanism is largely unknown. The physiological response to stress in the brain varies depending on the nature and duration of stress [5, 6], making a clear understanding of the pathogenesis of depression very difficult.
Previous reports have demonstrated that acute stress causes adaptive physiological and behavioral responses to maintain homeostasis, while prolonged stress exposure causes maladaptive responses, such as depression [7, 8]. A previous study demonstrated that restraint stress induces opposite alterations of the glucocorticoid receptor (GR) and mineralocorticoid receptor (MR) expression levels in various brain regions depending on the exposure period [9]. These two receptors are associated with the regulation of habitual coping behaviors to stressful conditions, as well as hypothalamic-pituitary-adrenal (HPA) axis activity, and is known as one of the endocrinological mechanisms against stress stimuli [10, 11]. Furthermore, Huang et al. [12] found that chronic unpredictable mild stress (CUMS)-induced changes in depression-related factors varied at different stages of depression in an animal model during stress exposure. These observations suggest that the effects of stress on depression-related factors are different depending on the time point of assessment. Therefore, it is important to investigate the dynamic changes in the features of depression to further understanding of the pathogenesis of depression.
In addition to restraint stress and CUMS, social defeat stress is also considered to be useful for assessing depression because the most important factor for the development of depression in humans is a psychological stress event such as threat or defeat [13, 14, 15, 16, 17]. In preclinical studies, socially defeated animals exhibit various profound physiological and behavioral changes that are reminiscent of the pathologies observed in human patients with depression [18, 19]. Thus, the social defeat model is useful to better understand the pathogenesis of depression. However, to our knowledge, previous studies on the pathological changes in the social defeat model were mostly based on observations at single time points after stress exposure; changes at different points during stress exposure were not dynamically observed.
Adult neurogenesis in the hippocampus is a process by which new granule cell neurons are added throughout life to the dentate gyrus (DG) of the hippocampus. Since the hippocampus is a brain region for regulating the emotion and stress response [20, 21], hippocampal neurogenesis dysfunction induced by stress exposure is an important hallmark for the development of depression [22, 23, 24]. Moreover, hippocampal neurogenesis plays a role in the regulation of the negative feedback of the HPA axis [25]. The HPA axis, when activated by stress, leads to corticotrophin-releasing hormone production in the hypothalamus that triggers adrenocorticotropic hormone release from the anterior pituitary and subsequent release of glucocorticoids (cortisol in humans and corticosterone [CORT] in rodents) from the adrenal cortex into circulation [10]. Neurogenesis impairment increases the stress response and blood glucocorticoid levels, and slows the recovery of HPA axis activity, which is associated with anxiety- and depression-like behaviors in rodents [25, 26]. Prolonged high levels of glucocorticoid leads to neuronal death or the failure of adult hippocampal neurogenesis in both humans and animal models of depression [27, 28, 29, 30, 31]. Moreover, numerous studies on social defeat stress have reported that stressed animals showed higher CORT levels, impairment of hippocampal neurogenesis, and increase in depression-like behaviors [32, 33, 34, 35, 36]. Based on the close relationship between hippocampal neurogenesis, HPA axis activity, and depression, it is necessary to dynamically observe these depression-related factors in social defeat models to better understand the pathogenesis of depression.
Our previous studies have demonstrated that an intermittent four-time exposure to social defeat stress using the resident/intruder paradigm caused impairments of hippocampal neurogenesis and affective behavior in male Sprague-Dawley rats [37, 38]. To reveal the dynamic changes in depression-related factors induced by social defeat stress in a stress-frequency-dependent manner, we exposed rats to social defeat stress one to four times. The different responses to these multiple social defeat stress exposures were evaluated in the socially defeated rats by examining depression-like behavior in the forced swimming test (FST), HPA axis activity response to acute stress in the stress reactivity test (SRT), and hippocampal neurogenesis.
A total of 23 male Sprague-Dawley rats (6 weeks of age at arrival; 160–190 g;
Kyudo Co., Ltd., Saga, Japan) were used in this study. They were individually
housed in plastic cages (23
The social defeat procedure in this study was adopted from a previous
publication [39]. Briefly, each session of social defeat stress was conducted for
30 min. Before the start of the social defeat procedure, the resident female
Long-Evans rat was removed, and the male was kept in the cage. A male
Sprague-Dawley rat, an intruder, was introduced into the cage of a resident.
Usually, the intruder rat is attacked by the aggressive resident male rats within
1–3 min and exhibits a submissive posture such as a supine posture and/or
freezing behavior, which indicates that the intruder has been psychosocially
defeated. Immediately after observing the defeat behaviors of the intruder, the
intruder was moved into a protective wire mesh cage (15
To test the reactivity of the HPA axis to acute stress, we performed the SRT
according to a previous study with slight modifications [40]. The SRT is
performed before and after the end of the social defeat stress period. All
animals were restrained for 15 min in an acrylic rodent restrainer (KN-325-B,
Natsume Seisakusho Co., Ltd., Tokyo, Japan). Immediately after the beginning (at
0 min) and at the termination (at 15 min) of restraint stress, blood samples were
collected from the same rat via the tail vein using a 23-gauge needle (01045,
Nipro, Osaka, Japan) and transferred into a tube containing 5
All animals were acclimatized for 1 week after arrival and subjected to an SRT (pre-SRT). Three days after the pre-SRT, animals were randomly assigned to five experimental groups. (1) Control group animals (N = 4) were intermittently placed in a novel cage four times, once every 24–48 h, over the course of 8 days. (2) One-time-defeated group animals (N = 5) were intermittently placed in a novel cage three times and subsequently exposed to social defeat once, every 24–48 h, over the course of 8 days. (3) Two-time-defeated group animals (N = 5) were intermittently placed in a novel cage two times and subsequently exposed to social defeat twice, once every 24–48 h, over the course of 8 days. (4) Three-time-defeated group animals (N = 4) were intermittently placed in a novel cage and subsequently exposed to social defeat three times, once every 24–48 h, over the course of 8 days. (5) Four-time-defeated group animals (N = 5) were subjected to intermittent social defeat four times, once every 24–48 h, over the course of 8 days. One day after the last stress session, all animals were subjected to post-SRT. One day after post-SRT, an FST was performed. Two hours after the FST, the rats were transcardially perfused with fixative for the evaluation of hippocampal neurogenesis. Social defeat stress, FST, and SRT were performed between 9:30 AM and 1:30 PM The experimental design is illustrated in Fig. 1.
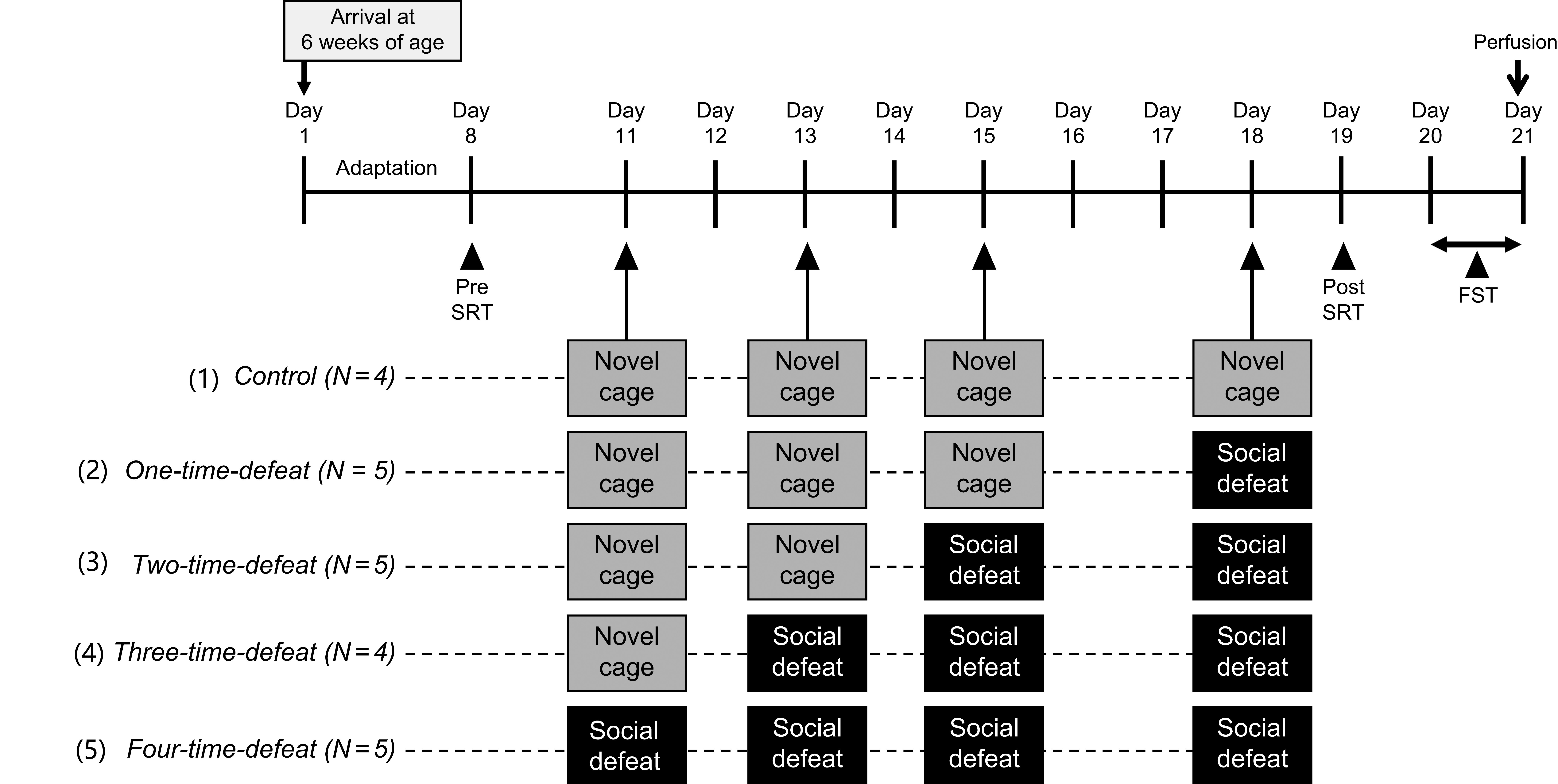
Experimental design. All animals were subjected to a stress reactivity test (pre-SRT) 3 days before the start of the stress sessions. In the stress sessions, a total of four bouts of stress exposures (novel cage stress and/or social defeat stress) were conducted. (1) Control group (N = 4) animals were intermittently subjected to novel cage stress four times. (2) One-time-defeated group (N = 5) animals were intermittently subjected to novel cage stress three times and then to social defeat stress one time. (3) Two-time-defeated group (N = 5) animals were intermittently subjected to novel cage stress two times and then to social defeat stress two times. (4) Three-time-defeated group (N = 4) animals were intermittently subjected to novel cage stress one time and then to social defeat stress three times. (5) Four-time-defeated group (N = 5) animals were intermittently subjected to social defeat stress four times. In all groups, on the day after the last stress session, SRT (post-SRT) was conducted again. One day after the post-SRT, animals were subjected to the forced swim test (FST), then perfused transcardially with a fixative to evaluate hippocampal neurogenesis.
The FST was conducted as previously described [39]. Briefly, each rat was forced
to swim in a Plexiglas cylinder, 20 cm in diameter and 50 cm in height,
containing water filled to a depth of 30 cm at 27
Two hours after the FST, sodium pentobarbital was used to deeply anesthetize the
rats. Then, they were perfused transcardially with saline followed by 4%
ice-cold paraformaldehyde in phosphate-buffered saline. The brain was resected
from the skull and post-fixed in the same fixative at 4 °C overnight. A
freezing microtome was used to cut 40-
The total number of DCX-positive cells was counted in all parts of the
rostrocaudal extent of the DG in the granular cell layer using a 40
Data were analyzed using StatView software Ver. 5 (Hulinks, Tokyo, Japan). The
data of each group were normally distributed (Shapiro–Wilk test), except for the
The effects of exposure to social defeat stress on the frequencies of
immobility, climbing, and swimming behaviors in the FST are shown in Fig. 2.
There were no significant changes in the frequency of swimming (Fig. 2, middle)
and climbing (Fig. 2, right-hand side) behaviors between the groups. In contrast,
one-way ANOVA indicated significant differences in the frequency of immobility
behavior (F
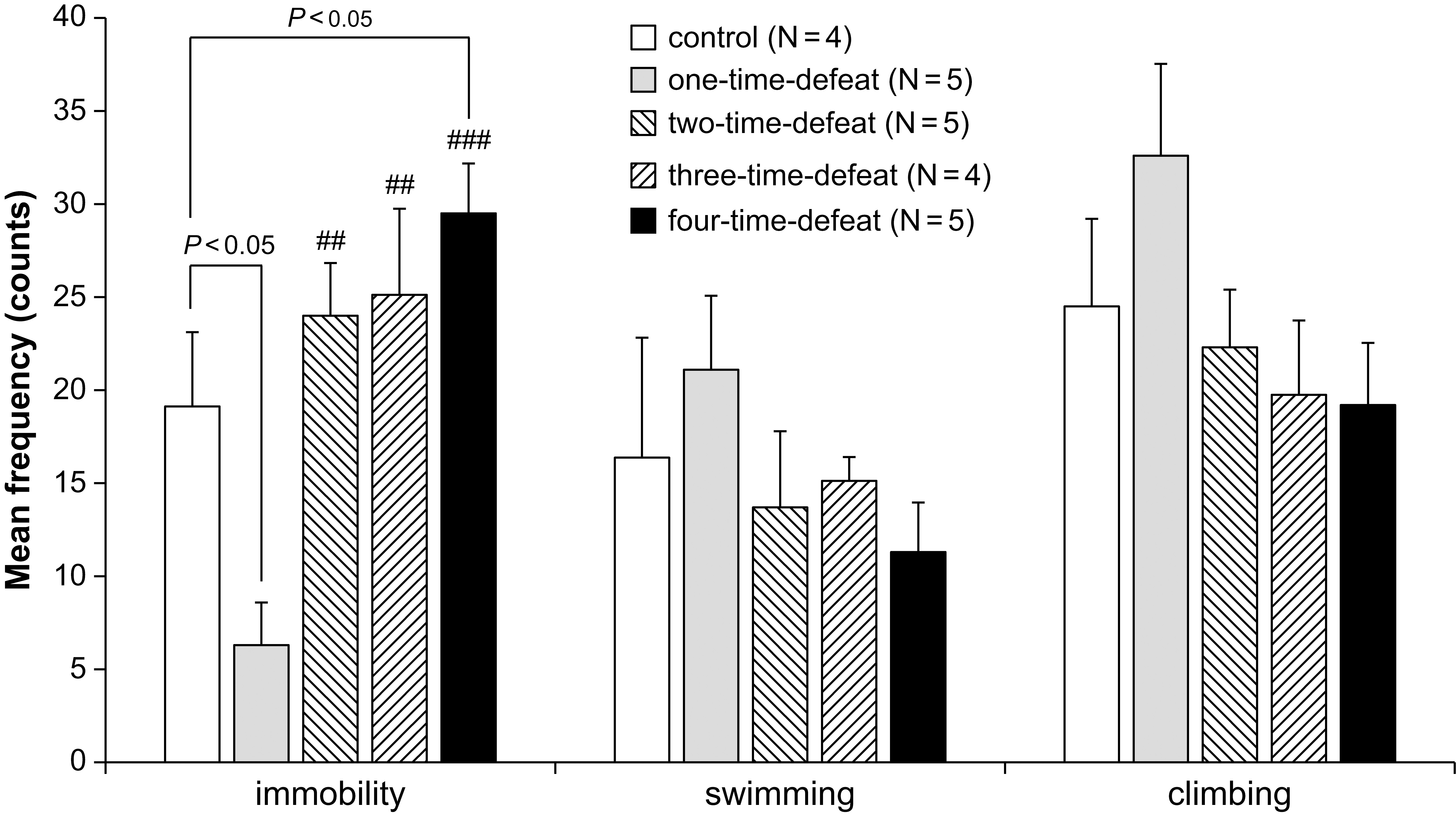
Effects of social defeat stress on animal behavior in the forced
swimming test. One-time social defeat stress significantly decreased immobility
compared with the control group in the forced swimming test, while four-time
social defeat stress significantly increased immobility compared with the control
group (left-hand side). Number of animals (N = 4–5) per group. Bars
represent the mean
The effects of exposure to social defeat stress on the changes in plasma CORT
levels in the SRT are shown in Fig. 3. We conducted the SRT both before the start
(pre) and after the termination (post) of the social defeat stress procedure to
examine the activity of the HPA axis response to acute restraint stress. At the
pre-SRT, there was no significant difference in the increase (
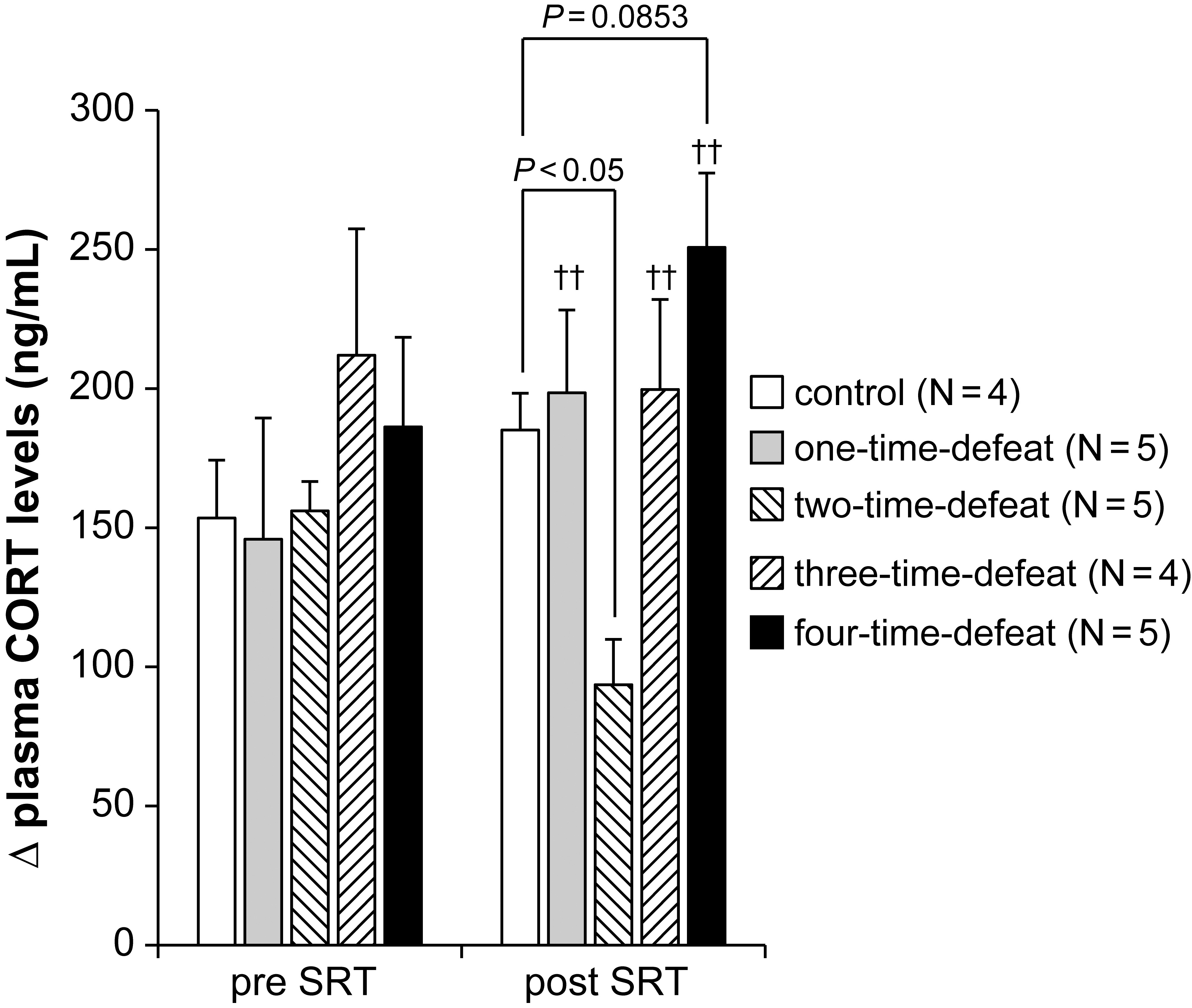
Effects of social defeat stress on
hypothalamic-pituitary-adrenal axis activity in the stress reactivity test. No
effect of social defeat stress was observed in the stress reactivity test
(pre-SRT, left-hand side). In contrast, social defeat stress significantly
decreased
However, the Kruskal–Wallis test revealed a significant difference between the
groups at the post-SRT (H = 11.266, p
Group | Δ Plasma CORT levels (ng/mL) | Pre- vs. post-SRT |
---|---|---|
Post-SRT – pre-SRT | p value | |
Control | 31.6 |
0.3203 |
One-time-defeat | 52.6 |
0.1380 |
Two-time-defeat | −62.5 |
0.0436* |
Three-time-defeat | −12.3 |
0.8792 |
Four-time-defeat | 64.5 |
0.0431* |
CORT, corticosterone; SRT, stress reactivity test.
All values are expressed as the mean
The number of DCX-positive cells in the DG resulting from multiple social defeat
stress is shown in Fig. 4. DCX-labeled cells were observed mainly in the
subgranular zone, which borders the hippocampal DG (Fig. 4a). Because of
technical constraints, brain samples for immunohistochemistry could not be
obtained from one animal in the two-time-defeated group. A separate analysis was
conducted on the dorsal and ventral parts of the DG, with the number of
DCX-positive cells in the subgranular zone expressed per unit volume of the
corresponding DG. No statistically significant difference was observed in the
density of DCX-positive cells in the dorsal DG of the hippocampus (Fig. 4b,
left-hand side). Contrastingly, one-way ANOVA indicated a significant difference
in the number of DCX-positive cells in the ventral hippocampus (F
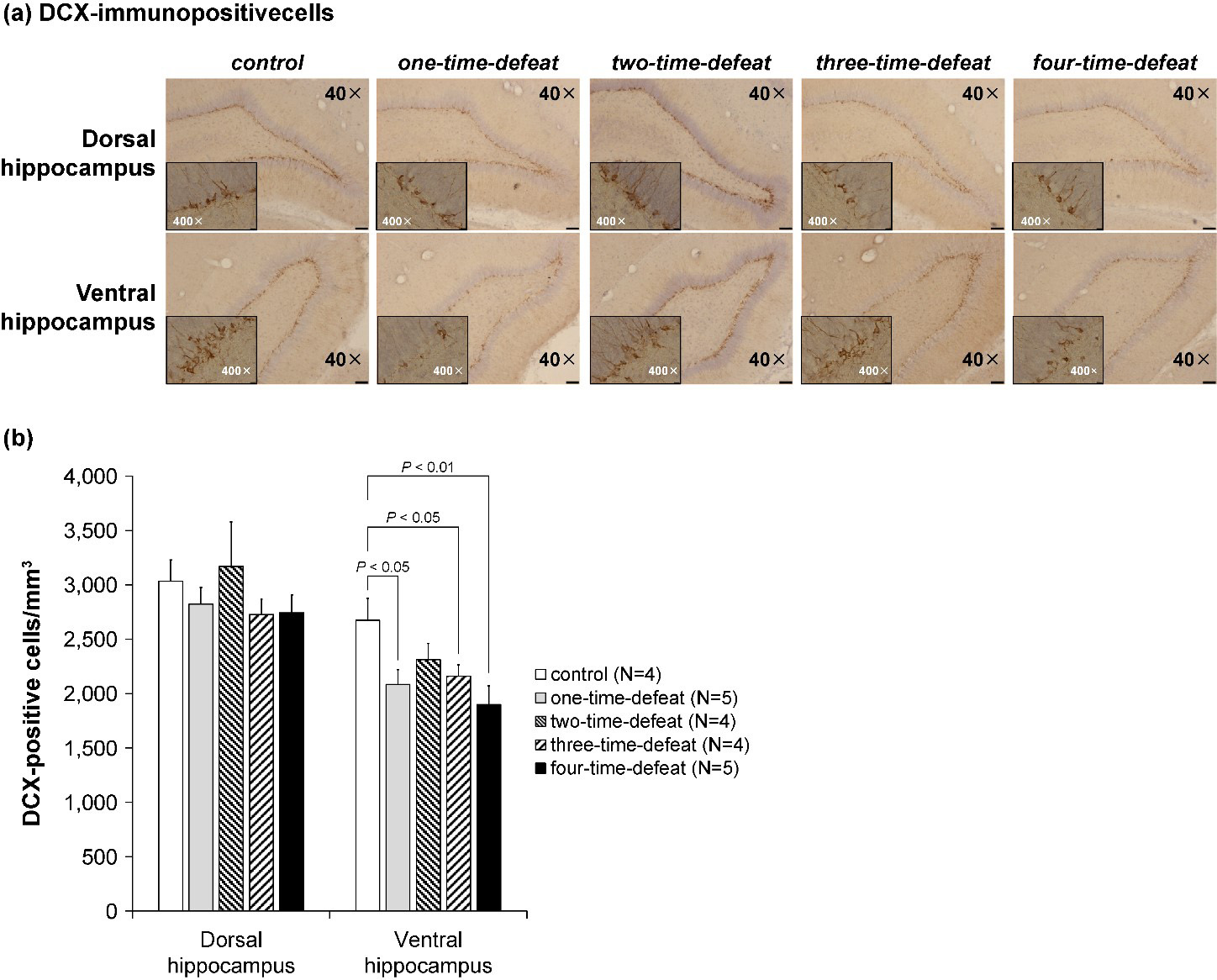
Photomicrographs of representative doublecortin
(DCX)-immunopositive cells in the dorsal and ventral hippocampal dentate gyrus of
the rats in the five groups (a; magnification:
In the current study, we assessed immobility behavior in the FST, activity of the HPA axis response to acute restraint stress, and the number of immature neurons in the DG of the hippocampus in rats subjected to different frequencies of social defeat stress exposure. We found that one or two stress exposures caused a decrease in immobility behavior, HPA axis activity, and the number of immature neurons. In contrast, immobility behavior and HPA axis activity of stressed rats increased with four stress exposures, but the number of immature neurons was decreased. These results suggest that social defeat stress produces dynamic changes in depression-related factors in a stress frequency-dependent manner.
We also found that social defeat stress using the resident-intruder paradigm induced opposite alterations in immobility behavior in the one-time- vs. four-time-defeated groups. One-time-stress exposure significantly decreased immobility, while four-time-defeated rats exhibited significantly increased immobility behavior. The FST is one of the most commonly used tests to assess behavioral despair or depression-like state in rodents [42, 43]. Chronic exposure to social defeat stress increases the duration of immobility, which can be decreased by antidepressant treatment [17, 44, 45, 46]. Thus, our results suggest that one-time social defeat stress exposure causes antidepressant-like effects, while four-time-stress exposure leads to a depressive state. In particular, the positive (shortening) effect of acute social defeat stress on immobility behavior in the FST is a particularly important result while considering that psychosocial stress is a common risk factor for the development of depression.
Regarding the depressive phenotype induced, we confirmed that rats who underwent
four-time-social defeat stress showed slightly increased HPA axis activity in the
post-SRT (p = 0.0853) and significantly increased
As mentioned above, hippocampal neurogenesis has been implicated in the regulation of depression-like behavior and the normalization of HPA axis activity after stress [25, 47, 53]; however, one-time stressed rats did not show an increase in depression-like behavior and HPA axis hyperactivity despite a decrease in neuroblasts/immature neurons in the hippocampus. One possible explanation for this discrepancy is that the impact of stress-induced increases in CORT levels on hippocampal functions may differ in a time-dependent manner.
Glucocorticoid receptors (GR and MR) are highly expressed in the hippocampus. Moreover, as neural stem cells in the DG of the hippocampus are located in close vicinity to blood vessels [54], stress and stress-induced elevated CORT levels may affect hippocampal functions and neurogenesis in the DG [30, 55]. Acute stress-induced increase in CORT has a positive effect on hippocampus-dependent memory consolidation, whereas chronic stress and prolonged exposure to elevated CORT levels have been associated with deficits in learning, memory, and retrieval [56, 57, 58, 59]. These findings support the possibility that acute and chronic social defeat stress may produce positive and negative effects on hippocampal functions, including negative feedback regulation of the HPA axis, respectively.
Regarding the positive effect of stress exposure on hippocampal neurogenesis, acute immobilization stress increases cell proliferation in the DG of the hippocampus [60]. In addition, acute glucocorticoid treatment enhanced phosphorylation of tropomyosin receptor kinase B and extracellular signal regulated kinase 1/2 in hippocampal neurons [61, 62], which are key mediators of increasing neurogenesis and the therapeutic response to antidepressants [63, 64]. Several studies have demonstrated that fluoxetine, an antidepressant, accelerates the maturation of immature neurons by shortening the immature stage of newborn cells, such as the DCX-expressing time window in the hippocampus [65, 66]. Thus, it can be speculated that one-time (acute) social defeat stress increases the maturation of newborn neurons, presumably shortening the DCX-positive stage and thereby lowering the number of cells at this stage at the time of analysis. The enhanced and accelerated hippocampal neurogenesis, induced by acute social defeat stress, may induce antidepressant-like behavior (decreased immobility in the FST) and normalization of HPA axis activity (a hippocampal function), whereas prolonged stress exposure, such as the four-time-defeated group in this study, may cause depressive behavior and HPA axis hyperactivity by decreasing hippocampal neurogenesis.
Notably, we observed interesting results in the two-time- and
three-time-defeated groups. First, the two-time-defeated rats had a significant
decrease in HPA axis activity compared with the other groups under the post-SRT
condition (Fig. 3, right-hand side). Experiencing social defeat stress twice
decreased the
Since this is a novel aspect of depression-related change induced by social defeat stress that has never been studied, the precise mechanisms are not known. However, a previous study showed that glucocorticoid pretreatment diminished the subsequent restraint stress-induced adrenocorticotropic hormone and CORT release in rats by GR occupancy in the hippocampus, the primary negative feedback mediator of the HPA axis [67]. Furthermore, several studies on social defeat stress have reported that plasma CORT levels were increased immediately after stress exposure, and repeated social defeat stress induced higher plasma CORT levels for a prolonged period after the last stress session [34, 36, 52, 68]. These observations suggest that two bouts (repeated) of social defeat stress elevated plasma CORT levels for a long duration in the hippocampus, similar to a CORT pretreatment condition that may suppress the activity of the HPA axis response to restraint stress in the two-time-defeated group. Subsequently, hypoactivity of the HPA axis may offset the positive effects of CORT on hippocampal neurogenesis and immobility behavior that appeared in the one-time-defeated rats.
Next, the three-time-defeated rats had a decreased number of immature neurons in the hippocampus, but immobility and activity of the HPA axis were comparable to those of the control rats in this study. We speculate that the reduction of immature neurons resulted from the increase in stress exposure frequency because four bouts of social defeat stress led to a depression phenotype. Hill et al. [69] showed that a certain level of neurogenesis is required for exerting its functions. Based on this, it is possible that three bouts of stress led to a prodromal phase of depression during which the decrease in adult-generated neurons that functionally integrate into hippocampal circuits is not at a level that causes functional deficits. To obtain precise data on hippocampal neurogenesis to further support our hypothesis, future studies should assess the ratio of DCX-positive cells to Ki-67-positive cells, which is useful as a proxy for cell maturation speed and survival [70], and use other maturation markers, such as NeuN, PSD95, and calbindin, and the dendritic complexity of DCX-positive cells should be quantified. Additionally, GR and MR are densely expressed by granule cell neurons in the DG of the hippocampus and play a crucial role in HPA axis regulation and depression [71, 72, 73]. A previous study demonstrated that restraint stress dynamically changed GR and MR expression levels in various brain regions, including the hippocampus, depending on the exposure period [9]. For instance, acute restraint stress induced an increase in hippocampal MR protein levels and decrease in hippocampal GR protein levels. Conversely, repeated restraint stress downregulated hippocampal MR protein levels [9]. Therefore, we plan to examine the effects of social defeat stress on hippocampal GR and MR levels at varying frequencies of stress exposure in the future.
A recent study demonstrated that a CUMS-induced animal model of depression exhibited dynamic changes in depression-related features at different stages after the onset of depression [12]. Thus, to our knowledge, the present study is the first to show the dynamic changes in depression-related features in a social defeat animal model at the preclinical stage of depression (the stage from the start of stress exposure to the development of depression).
However, our study has several limitations. First, considering that the number of rats in each group was low (N = 4–5), caution should be exercised when interpreting the results or drawing conclusions. Therefore, further statistical analyses in a future study with sufficient sample sizes are warranted. Second, we used only the FST to assess depression-like behaviors. Thus, future studies on the dynamic effects of social defeat stress on other depression-related behaviors should use additional tests, such as the sucrose preference test and tail suspension test. Third, we used only male rats, which are highly motivated to defend their territory against unfamiliar males. The resident/intruder paradigm cannot be used with female rodents since they do not demonstrate territorial behaviors [14]. However, in humans, women are two to three times more likely to develop depression than men [74]. Therefore, future studies should assess stress-induced dynamic changes in depression-related factors in a stress frequency-dependent or duration-dependent manner in female rats using a different stress paradigm, such as CUMS.
In summary, our data showed that social defeat stress, using the resident/intruder paradigm, could produce a biphasic effect on symptomatic features of depression, such as behavioral despair, HPA axis activity, and hippocampal neurogenesis, in a stress-frequency-dependent manner. Acute social defeat stress (one-time-defeat) enhances a coping response to stressful conditions, whereas repeated stress exposure (four-time-defeat) leads to continuous maladaptive stress responses, possibly breaking down to a chronic psychopathological disease state of depression.
The datasets used and analyzed during the current study are available from the corresponding author on reasonable request.
HH, MM, YM, KO, and ME designed the study. HH, MM, and SK acquired the data, and HH, MM, YM, KT, and TM analyzed the data. HH and MM wrote the article, which all authors reviewed and approved for publication.
We performed all animal care and use procedures under the regulations established by the Experimental Animal Care and Use Committee of Fukuoka University, which follow universal principles of laboratory animal care (Aug. 24, 2015; approval number: 1508860).
We would like to thank Editage (https://www.editage.com/) for English language editing.
This work was supported in part by the Japan Society for the Promotion of Science (JSPS) KAKENHI Grant Number 20K16657.
The authors declare no conflict of interest.
Publisher’s Note: IMR Press stays neutral with regard to jurisdictional claims in published maps and institutional affiliations.