Academic Editor: Irene Messina
Background: The overconsumption of a high-fat diet (HFD) has been repeatedly blamed as being a possible contributor to the global prevalence of emotional problems in modern society. Our group recently demonstrated the deleterious effect of a chronic HFD throughout adulthood on both emotional behavior and neuroplasticity markers in mice. As a heightened preference for palatable HFDs from the time of the juvenile period (when the brain is particularly vulnerable to environmental insults) is universal among populations around the world, a comparison of the consequences of chronic HFDs starting from juveniles or adults will assist in obtaining better knowledge of the impact that chronic HFDs have on mental health, thus potentially leading to the discovery of more effective strategies for reducing the incidence of psychiatric disorders. Methods: In the present study, male C57BL/6J mice with an initial age of 4 weeks (IA-4 W) or 8 weeks (IA-8 W) were separately assigned to two subgroups and fed either a control diet (CD, 10 kJ% from fat) or HFD (60 kJ% from fat) for 9 months followed by an analysis focused on metabolic, emotional behavioral, and neuroplastic profiles. Results: The results illustrated that, in addition to abnormal glucolipid metabolism and insulin sensitivity, mice on a chronic HFD exhibited increased levels of anxiety and depression-like behaviors and aberrant hippocampal neuroplasticity. When compared with IA-8 W mice, several changes indicating systemic metabolic disturbance and neurobehavioral disorder after chronic HFD consumption were aggravated in IA-4 W mice, accompanied by exaggerated impairments in hippocampal insulin sensitivity and neurogenesis. Conclusions: These results not only provide in vivo evidence that the juvenile stage is a critical period of vulnerability to detrimental effects of HFD consumption on metabolic and neuronal function but also suggest dampened hippocampal insulin signaling as a potential link between prolonged HFD consumption and negative neurobehavioral outcomes. Considering the substantial burden posed by psychiatric disorders and the high prevalence of HFD among youth, these observations are meaningful for raising awareness of the harmful effects of excessive dietary fat intake and developing strategy for preventing mental disorders.
Emotions play a central role in all individuals’ lives. Emotional disorders are debilitating and constitute a considerable worldwide health burden with profound social and economic consequences [1, 2]. Consistent evidence from clinical, epidemiological, and animal studies has indicated that excessive high-fat diet (HFD) consumption has adverse effects on emotional functions [3, 4]. However, the nature of the association between mental disorders and HFD is far from being elucidated. The juvenile period is a critical stage for brain development and a period when the brain is particularly vulnerable to multiple factors [5, 6]. Given the persistent prevalence of HFD consumption among juveniles and adults in modern society, it is worth investigating the neurobehavioral outcomes of prolonged HFD consumption beginning in juveniles or adults, as well as the underlying mechanisms.
A high-fat diet is a recognized risk factor for abnormal glucolipid metabolism, and a long-term high-fat diet will significantly increase the risks of a series of metabolic disorders, such as diabetes mellitus, which is characterized by insulin resistance [7]. Insulin receptors (IR) are widely expressed not only in peripheral muscles, liver, and adipose tissue but also within the central nervous system (CNS), including the hippocampus, which is a key brain region involved in cognitive and emotional functions [8, 9, 10]. Insulin in the bloodstream crosses the blood-brain barrier into the brain, where it binds to the IR and activates a series of insulin signaling pathways [11]. It has been widely demonstrated that insulin plays a critical role in hippocampal neuroplasticity via the insulin receptor signaling pathway [12, 13] and that an impaired insulin receptor signaling pathway in the brain has been repeatedly reported in subjects with neurological disorders [14, 15, 16]. Although a chronic HFD is well known to induce peripheral insulin resistance and emotional abnormalities [17], the idea of whether central insulin sensitivity is altered after a chronic HFD, as well as the relationship between central insulin sensitivity and neuroplasticity, remain somewhat controversial.
Neurogenesis and synapticplasticity, two important manifestations of hippocampal neuroplasticity, are essential biological basis for the regulation of cognitive/emotional functions in the hippocampus [18, 19]. N-methyl-D-aspartic acid receptor (NMDAR, a member of the ion channel glutamate receptor family) and brain-derived neurotrophic factor (BDNF) play important role in hippocampal neuroplasticity [18, 20]. Accumulating evidence indicates that synaptic NMDAR number and subunit composition are change dynamically during development and in response to life experience [21]. Several lines of evidence suggest an important role of the NMDAR in neuronal development, synaptic plasticity, learning, memory and emotional processing [22, 23]. BDNF, a member of the neurotrophic family which is involved in promoting synaptic efficacy, neuronal connectivity and neuroplasticity, is widely expressed in different brain regions including the hippocampus [24, 25]. BDNF is known to play a critical role in hippocampal neurogenesis [26], which is associated with depression in both rodents and humans [27]. There is increasing evidence that the insulin receptor signaling pathway in the hippocampus is involved in the biological processes of hippocampal BDNF expression, neurogenesis, and NMDAR expression and transport [24, 28, 29]. Therefore, it is imperative to investigate the alterations of hippocampal neuroplasticity after chronic HFD consumption and its contribution to the emotional-behavioral effects induced by chronic HFD.
The current study in male C57BL/6J mice evaluated the metabolic and neuronal behavioral effects of long-term (9 months) HFD intake starting from the age of 4 weeks (approximately equivalent to the human period of juvenile) or 8 weeks (approximately equivalent to young adult in human) and investigated the potential underlying mechanism through a series of investigations focused on hippocampal neuroplasticity and the insulin receptor signaling pathway.
To avoid sex-dependent differences, we included only male mice in the study. SPF-grade healthy male C57BL/6J mice were provided by Nanjing University-Nanjing Institute of Biomedical Research (license number: SCXK[Su]2015-0001). A clean-level feeding environment with humidity was provided by the Southeast University Medical Laboratory Animal Center, with standard conditions (7 AM to 7 PM light cycle, 22 °C temperature, 55% humidity).
After one week of adaptive feeding, C57BL/6J mice at the initial age of 4 weeks
(IA-4 W) and 8 weeks (IA-8 W) were separately subrandomized into a CD group (fed
a control diet (CD): TP23303 (for juvenile mice, 3.5 kcal/g, 10% of energy from
fat, 19.4% from proteins, and 70.6% from carbohydrates) and/or TP23403 (for
adult mice, 3.5 kcal/g, 10% of energy from fat, 14.1% from proteins, and 75.9%
from carbohydrates)) and a high-fat diet group (fed a high-fat diet (HFD):
TP23300 (for juvenile mice, 5.0 kcal/g, 60% of energy from a lard and soybean
oil mixture contain a lard/soybean oil ratio of about 10:1, 19.4% from proteins,
and 20.6% from carbohydrates) and/or TP23400 (for adult mice, 5.0 kcal/g, 60%
of energy from a lard and soybean oil mixture contain a lard/soybean oil ratio of
about 10:1, 14.1% from proteins, and 25.9% from carbohydrates)), respectively.
The mice were housed in regular polycarbonate plastic cages (29 cm (length)
As shown in the schematic diagram of the experimental setting (Fig. 1A), upon completion of the dietary treatment, all of the mice underwent behavioral testing followed by tissue collection. All of the animal procedures were conducted with ethical permission and in accordance with the University Committee for Laboratory Animals of Southeast University, China. Technicians involved in all tests were blinded to the assignment of groups to all samples.
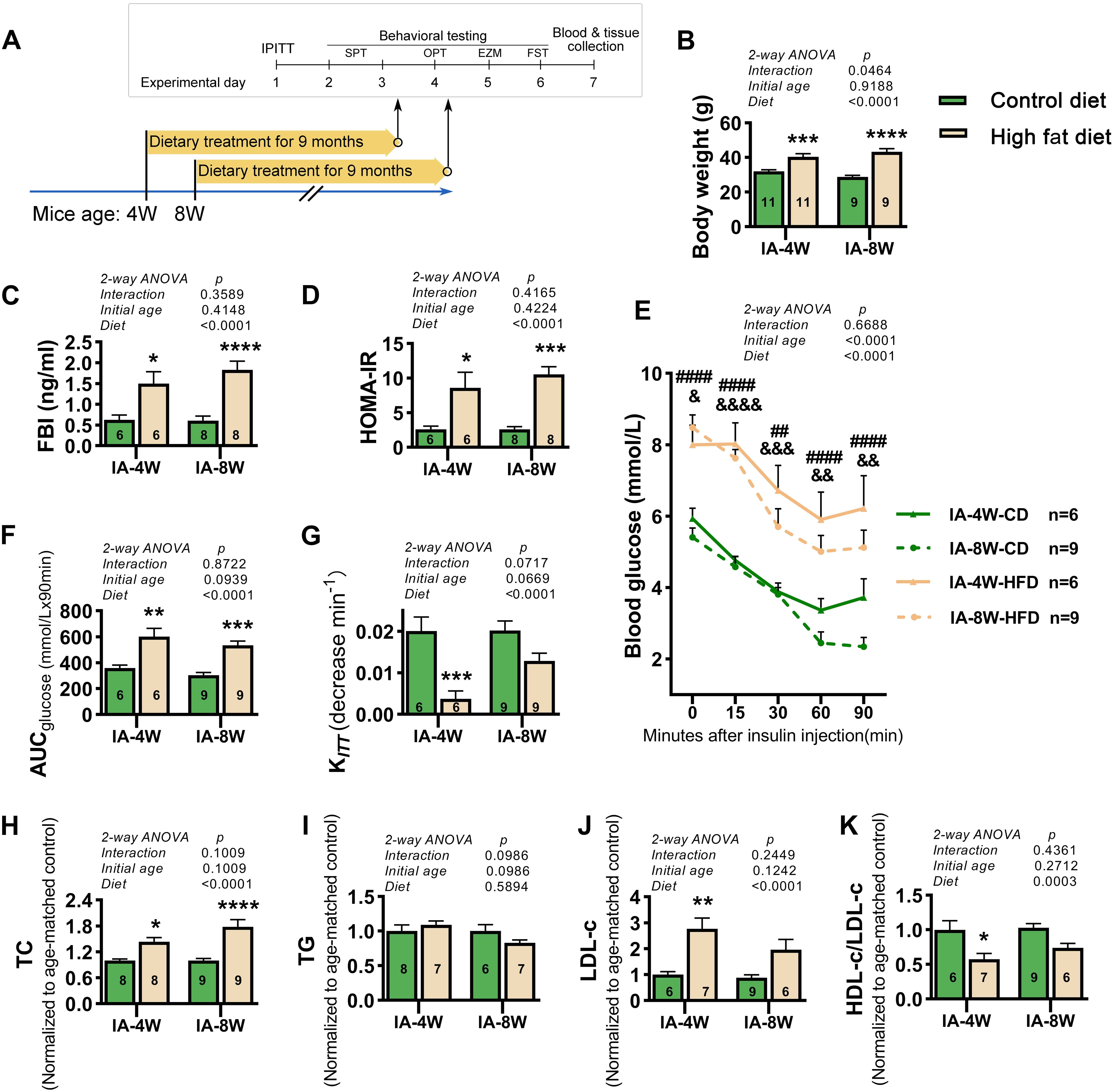
Effect of HFD consumption on metabolic and serum biochemical
parameters. (A) Schematic overview showing the experimental grouping, the
duration of dietary treatment, and time of behavioral testing and tissue
collection. (B–K) The values of body weight (B), fasting blood insulin (FBI, C),
the homeostasis model assessment of insulin resistance (HOMA-IR, D), blood
glucose levels that were recorded during the IPITT (E), the area under the blood
glucose curve during IPITT (AUCglucose, F), the blood glucose reduction rate
(K
Upon completion of the 9-month dietary treatments as designated, the
intraperitoneal insulin tolerance test (IPITT) on 4-h-fasted mice was initiated
at 9:00 AM. After measurement of body weight (BW), blood was collected from the
tail vein just prior to (0 min) and at 15, 30, 60, and 90 min after an
intraperitoneal injection of insulin at a dose of 0.75 U/kg body wt. A Bayer
Contour glucose monitor (Bayer HealthCare LLC, Whippany, NJ, USA) was used for
the measurement of blood glucose levels. The blood glucose level before insulin
loading (0 min) were also taken as fasting blood glucose level (FBG). IPITT
results were expressed as both a time course of absolute blood glucose
measurements and the area under the curve (AUCglucose). As a widely used insulin
tolerance test (ITT) derived index of in vivo systemic insulin
sensitivity, the blood glucose reduction rate (K
After the behavioral testing, blood was collected for the assessment of triglycerides (TG), total cholesterol (TC), low-density lipoprotein cholesterol (LDL-C), and high-density lipoprotein cholesterol (HDL-C) by using mouse-specific kits (Cat#: A110-1, A111-1, A112-1, and A113-1) purchased from the Nanjing Jiancheng Bioengineering Institute (Nanjing, China).
All behavioral assessments were performed during the light phase following a 1 h habituation to the test room before each test. Behavioral tests were conducted in the following sequence: sucrose preference test (SPT), open field test (OFT), elevated zero maze (EZM), and forced swim test (FST). The animals were handled for at least 3 min each for 5 consecutive days before the initiation of the behavioral tests. The behavioral apparatus was cleaned with 70% ethyl alcohol between each individual test. All of the behavioral tests were recorded on video and analyzed with a digital tracking system (Super Maze, Shanghai Xinruan Information Technology Company, Shanghai, China).
Sucrose preference test (SPT), a widely used measure of the ability to
experience pleasure as a feature of clinical depression (anhedonia) [33], was
performed as previously described by using a two-bottle testing paradigm with a
water and 1% sucrose solution [34]. Training (with both bottles) began one day
prior to treatment, and testing continued for 24 h after treatment. The sucrose
preference (SP) value was calculated as SP (%) = sucrose consumption/(sucrose
consumption + tap water consumption)
The OFT is used to evaluate the autonomous behavior and exploratory habits of
experimental animals in novel environments [35]. The open-field box is an opaque
box with a size of 40
EZM examines the animals’ anxiety/depression states by exploiting their exploratory nature of new environments and their fear of high open arms to develop conflicting behaviors [36]. The diameter of the circular runway in this device is 45 cm, the width is 6 cm, the runway consists of a pair of open-arm and closed-arm areas, and the closed-arm area consists of an inner wall and an outer wall 10 cm above the runway. The mice were placed in a closed arm area, and the activity of the mice was recorded for 5 min. The duration of movement of the mice in the open and closed arms and the number of times that the mice entered each arm were recorded.
For the FST, which is one of the most commonly used assessments of
depression-like behavior in animals [37], mice were individually placed in
transparent cylinders (18.5 cm height, 13 cm diameter) filled with 23
One day after the last behavioral test, the overnight-fasted animals were
weighed and deeply anesthetized with pentobarbital (100 mg/kg, i.p.). After blood
collection via cardiac puncture, the animals were transcardially perfused with 20
mL of 0.9% saline followed by 20 mL of 4% paraformaldehyde (PFA) in 0.1 M PBS.
For immunohistochemistry, the brains were then quickly excised, postfixed in 4%
PFA at 4 °C, cryoprotected in 30% sucrose in PBS until they sank, and
embedded in optimal cutting temperature (OCT) compound. Serial sagittal sections
(40-
Briefly, 8 uL of previously dispensed sample protein was subjected to 10%
SDS‒PAGE under reducing conditions and transferred to polyvinylidene fluoride
membranes (Immobilon PVDF, 0.45
Thereafter, enhanced chemiluminescence was used, and the images were exposed by
using a gel imager and then digitized. The protein expression of the target
protein strips was semiquantitatively analyzed by using ImageJ software (National
Institutes of Health, Bethesda, MD, USA); specifically, the integrated gray values of the
target protein strips were normalized by using the integrated gray values of the
internal reference protein strips (insulin/
Hippocampal neurogenesis was determined via immunohistochemistry as previously
described [38]. Every fifth sagittal section (200-
The data were analyzed by using SigmaPlot 12.0 for Windows (Systat Software
Inc., San Jose, CA, USA) and GraphPad Prism 8 (GraphPad Software, San Diego, CA, USA). All of the
values are expressed as the mean
Compared with the age-matched CD mice, HFD mice showed significant increases in
BW, FBI, HOMA-IR, and TC levels (Fig. 1B–D,H), as well as significantly higher
blood glucose levels and larger AUCglucose during IPITT (Fig. 1E,F), thus
illustrating the adverse effects of prolonged HFD consumption on glucolipid
metabolism. The negative glucose metabolic effects of chronic HFD were worse in
the IA-4 W group, although the duration of dietary treatment was identical, as
indicated by the clear (barely fail to reach significant) diet
As shown in Fig. 2A, HFD mice displayed significantly decreased sucrose preference during the SPT, indicating the inability to experience pleasure from enjoyable activities (a core symptom of depression in humans) [33].
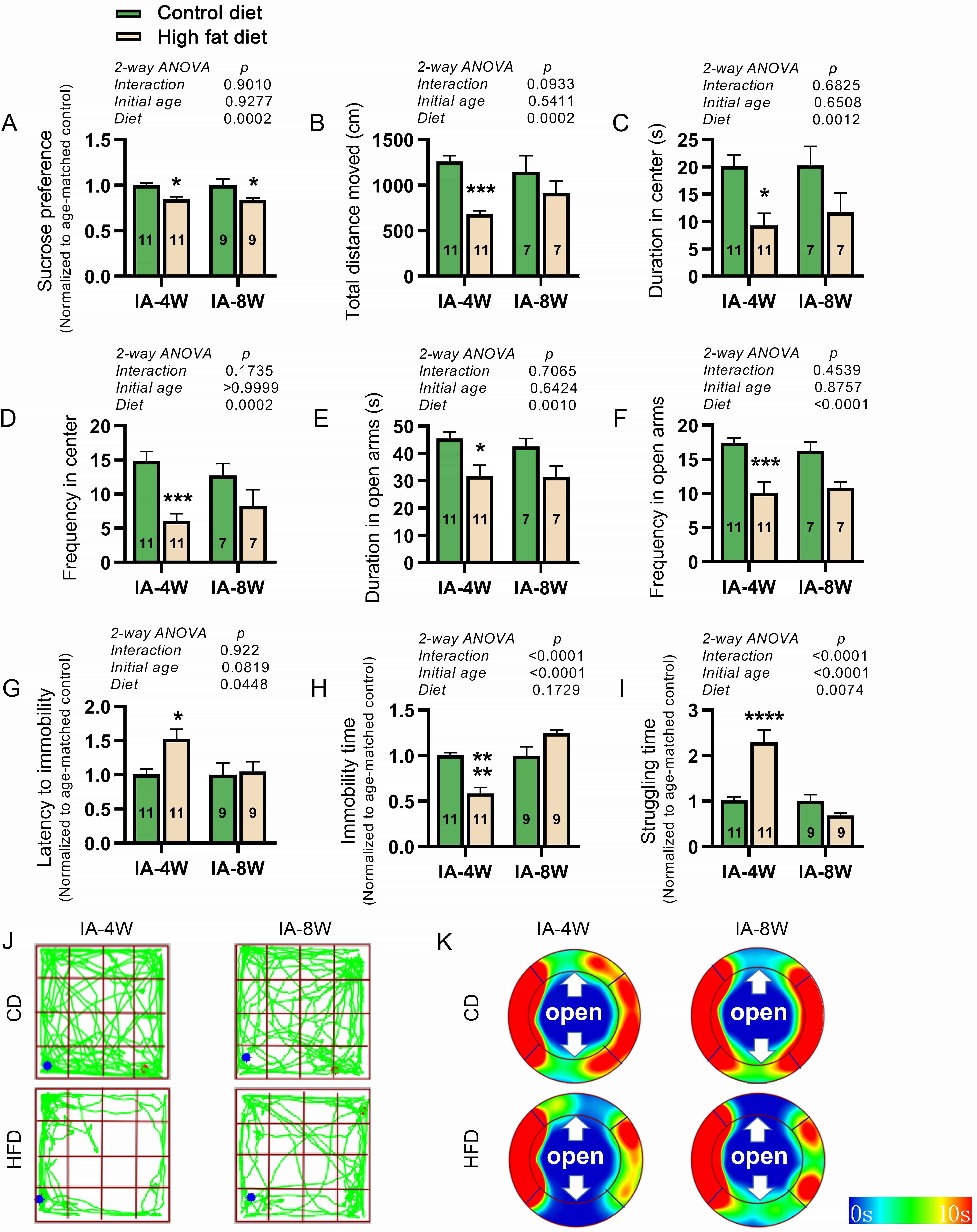
Effect of HFD consumption on emotional behavior in mice. (A)
Sucrose preference in SPT. (B–D) Total distance moved (B), duration in the
center (C), and frequency of center entries (D) in OFT. (E,F) Duration in open
arms (E) and frequency of open arm entries (F) in EZM. (G–I) Latency to
immobility (G), immobility time (H), and struggling time (I) during FST. (J)
Representative movement traces of mice from the CD and HFD groups in the OFT (the
green line is the trajectory; the blue dot is the starting point; the red dot is
the ending point). (K) Representative trajectory heatmap of mice from the CD and
HFD groups in the EZM. Values are shown as the mean
In the OFT, significant negative effects of prolonged HFD on the total traveling distance, the central duration, and the central entries were observed (Fig. 2B–D,J), whereas the significant difference between age-matched groups was only found in IA-4 W mice in post hoc tests (Fig. 2B–D). In the EZM (Fig. 2 E–F,K), HFD significantly decreased the duration in open arms (Fig. 2E) and frequency in open arms (Fig. 2F), while the post hoc tests revealed that the significant differences between age-matched group were only exhibited in the IA-4 W mice. These observations not only illustrated that prolonged HFD consumption decreased motor activity and exploratory behavior, as well as contributing to anxiety-like behavior, but also suggested that the anxiogenic effect of prolonged HFD consumption might be exacerbated by earlier exposure.
In the FST, HFD significantly increased the latency to immobility (Fig. 2G) and
struggling time (Fig. 2I). A significant diet
The key components of the insulin receptor signaling pathway in soleus muscle
and hippocampus after exogenous insulin loading were analyzed by WB (Fig. 3A,B).
In muscle, although no significant effect of diet on pY-IR/IR was shown (Fig. 3C), the values of pS-Akt/Akt were significantly decreased in HFD mice (Fig. 3D),
indicating impaired peripheral insulin signaling. In hippocampus, HFD
significantly increased the levels of insulin (Fig. 3E) but decreased the values
of pS-Akt/Akt (Fig. 3G), thus indicating dampened central insulin signaling. A
significant diet
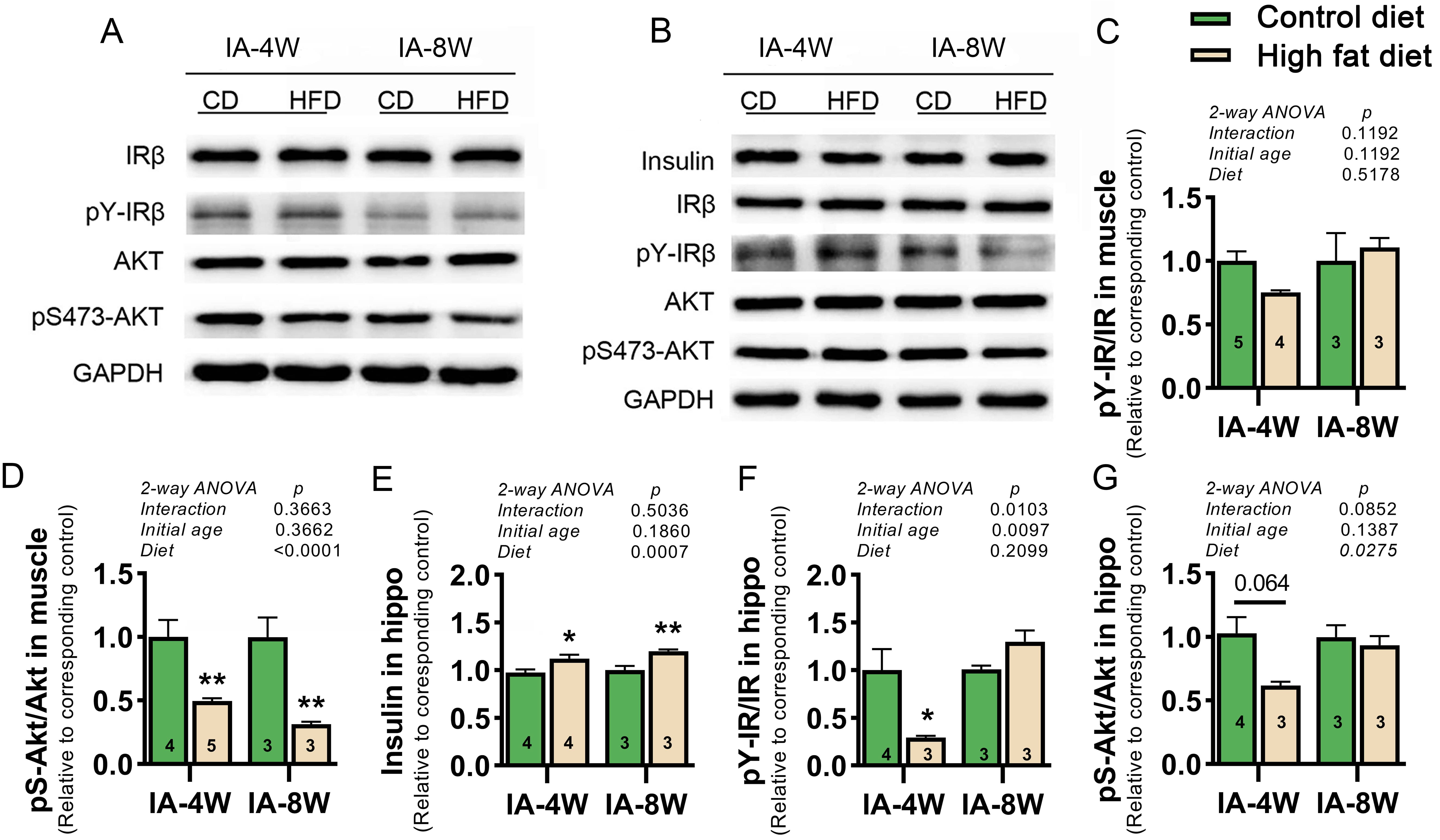
Effect of HFD consumption on the insulin receptor signaling
pathway in the soleus muscle and hippocampus. (A,B) Representative images of
Western blot (WB) analysis of key molecules of the insulin receptor signaling
pathway and internal reference GAPDH in the soleus muscle tissue (A) and
hippocampal tissue (B) of mice, respectively. (C–G) Graphs showing the levels of
pY-IR/IR (C) and pS-Akt/Akt (D) in the muscle, the levels of insulin (E),
pY-IR/IR (F), and pS-Akt/Akt (G) in the hippocampus. Values (shown as the mean
Fig. 4A shows representative images of DCX immunofluorescence labeling in the
dorsal and ventral DG regions of the hippocampus in mice after 9 months of diet
treatment. HFD significantly reduced the number of DCX-positive (DCX
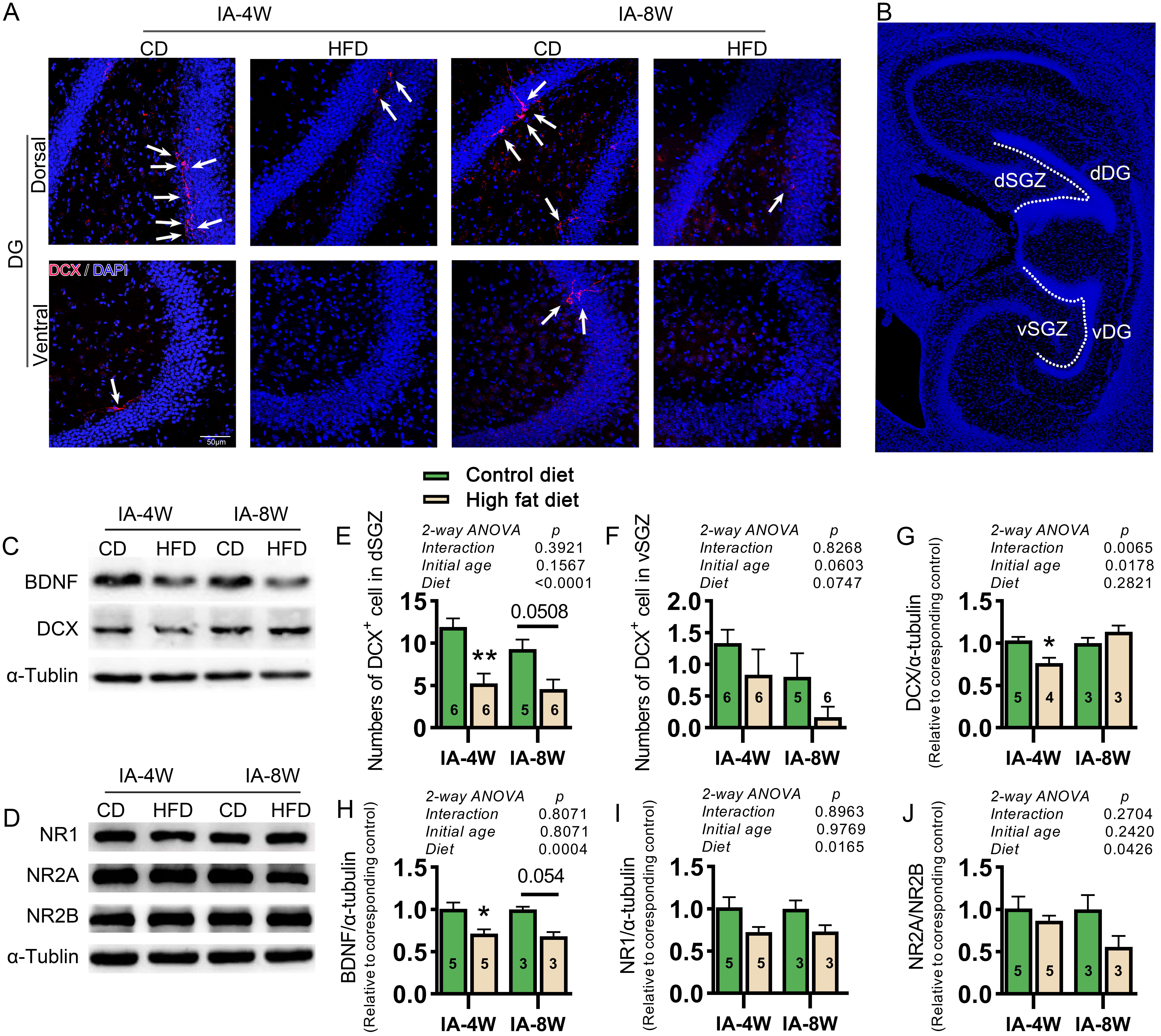
Effect of HFD consumption on neuroplasticity in the
hippocampus. Representative images of DCX immunofluorescence labeling in the
dorsal and ventral DG regions of the hippocampus in each group of animals (A),
image of a sagittal brain section with DAPI staining (B), representative images
of WB results of DCX and BDNF and NMDAR subunits and internal reference
Consistent with the results that have been previously reported [42], the present
study observed that mice on a chronic HFD exhibited overall insulin resistance,
hyperglycemia, hyperinsulinemia, and abnormal lipid metabolism. Furthermore, the
alterations in K
Emotional disorders have been found to frequently be associated with metabolic disorders and/or poor eating habits [43], thus suggesting a strong link between dietary structure and emotional problems. In the present study, the HFD mice exhibited depression-like behavior in the SPT and anxiety-like behavior in the OFT and EZM. Furthermore, compared with that of IA-8 W mice, the anxiogenic effect of prolonged HFD consumption was more pronounced in IA-4 W mice. Most intriguingly, in FST, a widely used behavioral paradigm to evaluate learned helplessness in response to the acute swim stressor, the IA-4 W-HFD mice exhibited a significantly longer immobility latency, shorter immobility time and longer struggle time, which were categorized as signs of irritability-like behavior rather than reduced depression, indicating an inappropriate coping response to uncontrollable stress [39, 40, 44]. Irritability is one of the main symptoms of depression, and it occurs frequently in the juvenile years, with patients being prone to intense emotional reactions, such as extreme anger and agitation, whenever they encounter stimuli or unpleasant situations, even if they are extremely mild [45]. Recent evidence has proposed that animals exhibiting irritability-like behavior in the FST are prone to exhibit extraordinarily anxious and stressful emotional behaviors [39, 46]. Additionally, studies have shown that depressed patients are at a significantly higher risk for suicide and that irritability can be an independent factor that increases the risk of suicide [47]. Our observation highlights the importance of a healthy diet from the juvenile stage to the improvement of quality of life across the remaining lifespan.
As mentioned above, our results illustrated that HFD mice developed obviously severe depressive-like behaviors, including anhedonia and behavioral despair, in the SPT. Furthermore, early exposure to HFD accentuated depression-like behaviors in mice, including reduced exploratory behavior in the EZM and reduced exploratory behavior in the OFT (which only occurred in HFD-IA-4 W mice). The reduction in the total distance traveled by HFD mice in the OFT may be due to the overweight-related lower motor activity, but it can also be interpreted as a sign of depression, including apathy, sadness, and anhedonia [48].
Insulin binds to the
The insulin receptor signaling pathway is thought to be important for neurogenesis, dendritic growth, neuronal survival, and synaptic plasticity [14]. The dysfunction of the hippocampal insulin receptor signaling pathway may lead to abnormal NMDAR expression and subunit composition, thus impairing hippocampal synaptic plasticity and neurogenesis [50]. Neurogenesis on the dorsal and ventral sides of the hippocampus is involved in regulating the cognitive and emotional functions of the organism, respectively. Neurogenesis in the DG region of the hippocampus occurs throughout the postnatal life course, and the rate of neurogenesis within the DG region can be altered under various physiological and pathological conditions [51]. The results of immunofluorescence in the present study showed that HFD induced a significant decrease in the number of DCX-positive cells in dorsal hippocampus. The DCX-positive cells in ventral hippocampus also lower in HFD mice compared with that of CD mice, while no significant difference was reached. As DCX-positive cells were generally seldom founded in ventral hippocampus of all mice tested in this study, we speculate that a floor effect might have masked the effects of HFD on ventral hippocampal neurogenesis. By WB analysis, the HFD induced significant decrease in hippocampal DCX was found in IA-4 W mice but not in IA-8 W mice. Differences in the methodology and the relatively small number of samples may explain the discrepancy between immunohistological data and WB data. The juvenile stage is the window of both vulnerability and opportunity for brain development [52]. This period may represent an optimal time for healthy lifestyle changes to have a positive and long-lasting impact on hippocampal neurogenesis [53]. In addition, the enhancement of hippocampal neurogenesis by modulating the structure of the diet in juvenile life may be a strategic option for the prevention of depression and cognitive decline [54]. In view of this, these results provide compelling evidence that the juvenile stage is a critical period when the vulnerability of neurogenesis to HFD is particularly higher.
Most suicidal individuals suffering from major depression have significantly reduced levels of BDNF in their brains [55]. Studies have shown that the direct infusion of BDNF into the hippocampus is sufficient to induce fast and long-lasting antidepressant effects [25]. In cultured hippocampal neurons in vitro, BDNF increased the number of NMDAR subunits on the plasma membrane of neurons [56]. BDNF has repeatedly been referred to as being a tremendous contributor to brain development and neuroplasticity by promoting neurogenesis, synaptic plasticity, and cell survival. It is essential for the survival of neurons in brain circuits that are involved in emotional and cognitive functions [24]. A previous study showed that the upregulation of the insulin receptor signaling pathway improves hippocampal BDNF expression and transportation, which further affects hippocampal neuroplasticity and alleviates depression [28, 57]. Alterations in the amount and subunit composition of NMDARs play an important role in the regulation of emotional behavior. In the present study, long-term HFD consumption induced a decrease in the levels of BDNF and NMDAR proteins, suggesting that the changes in hippocampal neurogenesis, BDNF expression and NMDARs composition may be involved in the long-term HFD feeding induced neuropsychiatric disorders.
There are some limitations to our study, which should be acknowledged. To avoid sex-dependent differences, we included only male mice in the study. Future research should be undertaken to explore sex-differentiated effects in metabolism, anxiety- and depressive-like behavior, and neuroplasticity. Another main limitation of this pilot study is the small sample size of groups, especially the low number of IA-8 W mice. Additional studies with a higher number of animals are needed to substantiate these findings.
This study demonstrated that juveniles may represent a critical period of vulnerability for metabolic and neuronal impact of HFD, and proposed dampened hippocampal insulin signaling as a potential link between the prolonged HFD consumption and the adverse neurobehavioral consequences. Our observations highlight the importance of healthy dietary structure from the juvenile period for improving emotional health in adults. Additional and more comprehensive methods will be needed in future studies to explore the emotional effects of HFD in juvenile as well as the underlying cellular mechanisms.
HFD, high fat diet; CD, control diet; IA-4 W, initial age of 4 weeks; IA-8 W, initial age of 8 weeks; IR, Insulin receptor; CNS, central nervous system; NMDAR, N-methyl-D-aspartic acid receptor; BDNF, brain-derived neurotrophic factor; IPITT, intraperitoneal insulin tolerance test; BW, body weight; FBG, fasting blood glucose level; ITT, insulin tolerance test; FBI, fasting blood insulin; HOMA-IR, homeostasis model assessment of insulin resistance; TG, triglycerides; TC, total cholesterol; LDL-C, low-density lipoprotein cholesterol; HDL-C, high-density lipoprotein cholesterol; SPT, sucrose preference test; OFT, open field test; EZM, elevated zero maze; FST, forced swim test; WB, Western blot; DCX, doublecortin; DG, dentate gyrus; SGZ, subgranular zone; IRS, insulin receptor substrate; AKT, protein kinase B.
LL designed the experiment and supervised the project. CY, DX, YX, QL, and XK managed the mouse cohorts. JZ, CW, and DX conducted the sample preparation for the histological study and WB. CS, CY, HZhang, and XY performed the histological procedures and data collection. CY, CW, and HZhang conducted Western blotting and data analysis. CY, HZhuang, YX, and LL were involved in the data interpretation. CY and LL contributed to the manuscript writing. All of the authors contributed to the article and approved the submitted version.
This study and included experimental procedures were approved by the institutional animal care and use committee of Southeast University (approval no. 20210302014). All animal housing and experiments were conducted in strict accordance with the institutional guidelines for the care and use of laboratory animals.
Support of confocal microscopy (OLYMPUS FV3000, Japan) from the Public Platform for Research Instruments, Southeast University School of Medicine, is gratefully acknowledged.
This work was supported by the National Natural Science Foundation of China (Grant Nos. 82171541, 81970893, 81670935) and the Jiangsu Provincial Key Laboratory of Critical Care Medicine (JSKLCCM-2022-02-002).
The authors declare no conflict of interest.
Publisher’s Note: IMR Press stays neutral with regard to jurisdictional claims in published maps and institutional affiliations.