Academic Editor: Rafael Franco
Background: Stem cells from human exfoliated deciduous teeth (SHED) are a mesenchymal stem cell type and have recently attracted attention for their high proliferative rate, multipotency, and immunosuppressive properties. However, SHED have not yet been investigated for anticancer properties. We therefore investigated whether SHED can be used as a treatment modality, particularly for anti-glioma therapy. Methods: In vitro, we examined the mobility of SHED and their ability to migrate towards glioma-conditioned medium and specific growth factors secreted by malignant gliomas. In vivo, we transplanted SHED into the left hemisphere of nude mice that had been previously implanted with human malignant glioma U87 cells into the right hemisphere. We assessed whether SHED had tumorigenic potential. Results: SHED exhibited strong migration ability towards malignant glioma in both in vitro and in vivo assays. In vitro, SHED migrated towards glioma-conditioned medium and specific growth factors such as stem cell factor, platelet-derived growth factor BB, C-X-C motif chemokine ligand 12, and vascular endothelial growth factor. SHED were accumulated around tumor cells in the contralateral hemisphere 1 week after transplantation. Moreover, SHED remained in the brains of nude mice 150 days after transplantation. Finally, we verified that SHED had no malignant transformation or engraftment of SHED in the mouse brain. Conclusions: Our findings indicate that SHED can potentially be applied to track malignant glioma.
Glioma accounts for approximately 20% of primary brain tumors. Recent advances in genomic analysis have made it possible to more accurately treat patients with effective chemotherapies. Additionally, the evolution of awake surgery, devices that improve tumor removal rates, and advances in radiation technology have remarkably improved clinical outcomes. Nevertheless, the 5-year survival rate for glioblastoma remains only 6.8% [1], and recurrences from any remnant tumor after treatment will affect prognosis [2]. Therefore, localized treatment is required to completely destroy all residual tumor cells, particularly any that have invaded the surrounding normal brain tissue. One promising approach to this is the development of delivery modalities that can bring antitumor agents to remnant tumor cells.
There are various strategies for localized glioma therapy such as photodynamic therapy, gene therapy, and oncolytic viral therapy. Of those, gene therapy is a good candidate to control localized tumor cells [3]. One of the gene therapy techniques that has recently been introduced is suicide gene therapy using the herpes simplex virus–thymidine kinase (HSV-TK)/ganciclovir (GCV) combination [4], or the cytosine deaminase/5-FC system [5, 6]. The key mechanism for these treatments is the so-called “bystander effect”, in which cancer cells are driven into apoptosis by genetically modified cells [4]. Several studies have investigated suicide gene therapy in recent years [7, 8, 9, 10]; however, a transporter that can carry the suicide gene to tumor cells and track malignant cells throughout the body has not been identified.
Stem cells are recognized to have the ability to migrate to tumors, and previous reports have introduced exogenous suicide genes into stem cells, and then allowed them to migrate to residual tumor cells for treatment [10, 11, 12]. This approach has also been attempted for malignant gliomas [13]. Neural stem cells (NSC), induced pluripotent stem (iPS) cells, multilineage-differentiating stress-enduring (Muse) cells, and mesenchymal stem cells (MSC) have all been shown to migrate to malignant gliomas [8, 9, 14], and the principle of tumor tropism has also been investigated in these contexts. Subsequent studies have confirmed that the growth factors, cytokines, and chemokines secreted by tumors attract stem cells [15, 16, 17].
Dental pulp stem cells (DPSC) are a type of MSC that exhibit several advantages over other types of stem cells. In vitro DPSC are easier than for other MSC because they have better growth properties [18, 19, 20, 21]. DPSC have been found to have the ability to differentiate into both mesoderm and ectoderm tissues [22]. Stem cells from human exfoliated deciduous teeth (SHED) are a type of DPSC and have similar proliferative potential as DPSC. SHED are more easily and noninvasively collected when deciduous teeth start falling out during childhood compared with DPSC [23]. SHED have been used for various clinical trials in the field of the regenerative therapy because of their increased proliferative capacity [24]. However, no report has verified whether SHED can be used as a vector for suicide gene therapy in an anticancer regimen for malignant glioma.
In this study, to investigate whether SHED could be used as a treatment modality for anti-glioma therapy, we examined the tropism of SHED for malignant gliomas in vitro and in vivo. Our findings confirmed the tumor tropism and safety of SHED, which may contribute to verifying whether modified SHED can be developed into a novel anti-glioma therapy.
SHED isolated from a 9-year-old girl’s mobile deciduous tooth were provided by Kidswell Bio Corporation (formerly Gene Techno Science Co., Ltd., Tokyo, Japan).
The specifications of SHED such as surface protein expression and multilineage
potential are available at
https://www.summitpharma.co.jp/japanese/service/s_ATCC_msc.html. Regarding
surface protein expression, SHED were positive for CD73, CD90, and CD105 and
negative for CD14, CD19, CD34, and HLA-DR. SHED were cultured in expansion medium
consisting of Minimum Essential Medium-alpha (Thermo Fisher Scientific, Waltham,
MA, USA) supplemented with 20% fetal bovine serum (FBS; Nichirei, Tokyo, Japan),
1% Penicillin–Streptomycin (10,000 U/mL; Thermo Fisher Scientific), and 100
The human glioma cell lines U87 and U251 were purchased from the American Type
Culture Collection (Manassas, VA, USA) and from the Japanese Collection of
Research Bioresources (Ibaraki, Osaka, Japan), respectively. The human glioma
cell line U87-luc (Bioware Ultra Cell Line U-87 MG-luciferase2) was purchased
from Caliper Life Sciences (Waltham, MA, USA). Cells were cultured in Dulbecco’s
Modified Eagle’s medium (DMEM) (Sigma-Aldrich, St. Louis, MO, USA) supplemented
with 10% FBS at 37 °C in a humidified atmosphere of 5% CO
Human fibroblasts derived from fetal skin (human dermal fibroblast: hDF;
RCB1139) were obtained from Riken BioResource Center (Tsukuba, Japan) and
cultured in DMEM supplemented with 10% FBS at 37 °C in a humidified
atmosphere of 5% CO
Human astrocytes were obtained from Lonza (Basel, Switzerland). Cells were
cultured in Astrocyte Growth Medium SingleQuots™ Supplements and
Growth Factors (Ready-to-use) (Lonza, Basel, Switzerland) at 37 °C in a
humidified atmosphere of 5% CO
To evaluate the migration, hMSC-AT, hMSC-BM, SHED, and hDF alone cells were incubated
in 60-mm dishes at 1
In vitro migration was examined using 24-well Matrigel Invasion
Chambers (Corning Discovery Labware, Bedford, MA, USA), which contained
8-
To determine the ability of SHED to migrate towards specific growth factors, the lower chamber was filled with 0.75 mL of DMEM and specific growth factors, including stem cell factor (SCF), platelet-derived growth factor BB (PDGF-BB), C-X-C Motif Chemokine Ligand 12 (CXCL12), and vascular endothelial growth factor (VEGF), (Abcam PLC, Tokyo, Japan), which were added at concentrations ranging from 0.1 to 100 ng/mL.
For experiments that involved blocking specific growth factors, U87 and U251 CM
were treated with anti-SCF, anti-PDGF-BB, anti-CXCL12, or anti-VEGF neutralizing
antibodies (Abcam PLC) at concentrations of 1 to 10
The following experiments were performed and supervised as per guidelines approved by the Animal Care Committee of the Hamamatsu University School of Medicine Animal Care Facility (approval number 2020089).
The mouse model of glioma was based on protocols used in previous reports [8, 30]. Briefly, female BALB/c slc nu/nu mice (18–22 g, 8–9-weeks-old, Nippon SLC,
Shizuoka, Japan) were subcutaneously injected with an anesthetic mixture
comprising 0.75 mg/kg medetomidine (Nippon Zenyaku Kogyo, Fukushima, Japan), 4.0
mg/kg midazolam (SANDOZ, Tokyo, Japan), and 5.0 mg/kg butorphanol (Meiji Seika
Pharma, Tokyo, Japan). The human glioma cells U87-luc (1
To monitor bioluminescence signals of U87-luc cells, XenoLight Rediject D-luciferin (Summit Pharmaceuticals International, Tokyo, Japan) was injected intraperitoneally at 150 mg/kg body weight, followed by subcutaneous injection of the anesthetic mixture. Then the mice were placed on a stage inside the camera box of the IVIS200 imaging system coupled with a cooled CCD camera (Caliper Life Sciences, Waltham, MA, USA) 20 min after D-luciferin injection. The detected light emitted from U87-luc cells was digitized and electronically displayed as a pseudo color overlay onto a grayscale image of the animal. Images and measurements of luminescence signals were analyzed with Living Image software version 3.0 (Caliper Life Sciences, Waltham, MA, USA) and quantified as photons per second [9]. Images and measurements of luminescence signals were acquired on day 5.
To evaluate the ability of SHED to target glioma cells in the brain and to
understand the relationship between the number of SHED that accumulated around a
glioma lesion and tumor size, we labeled SHED with ferucarbotran
(Resovist® Inc., Fujifilm, Tokyo, Japan), in which SPIO was used
to label the cell membrane with iron, and the number of SHED surrounding the
lesion at the maximum cross-sectional area were counted. SHED were counted within
the tumor and within 100
For tumor sections from the SPIO experiment, while the Prussian Blue might stain
macrophages, Qtracker probe (Thermo Fisher Scientific) was also used to evaluate
the in vivo migratory ability of SHED. Malignant glioma cells (U87-luc)
were transplanted into the right hemisphere of the mice (n = 3), and then SHED
were transplanted into the contralateral hemisphere. U87-luc cells (1
To use SHED as a transporter for gene therapy in malignant glioma patients, we
first assessed whether SHED had tumorigenic potential in vivo. SHED (5
The Excel Statistical Program File “ystat 2008.xls” (programmed by Shinya
Yamazaki, DDS, Ph.D., Igaku-Tosho-Syuppan, Tokyo, Japan, ISBN4-87151-351-3) was
employed for statistical analyses. All data are presented as mean
The mobility of SHED was compared with those of hMSC-AT and hMSC-BM in scratch
assays, with hDF used as a control. The percentages of cells that moved into the
scratch range were calculated as follows: SHED, 31.2%
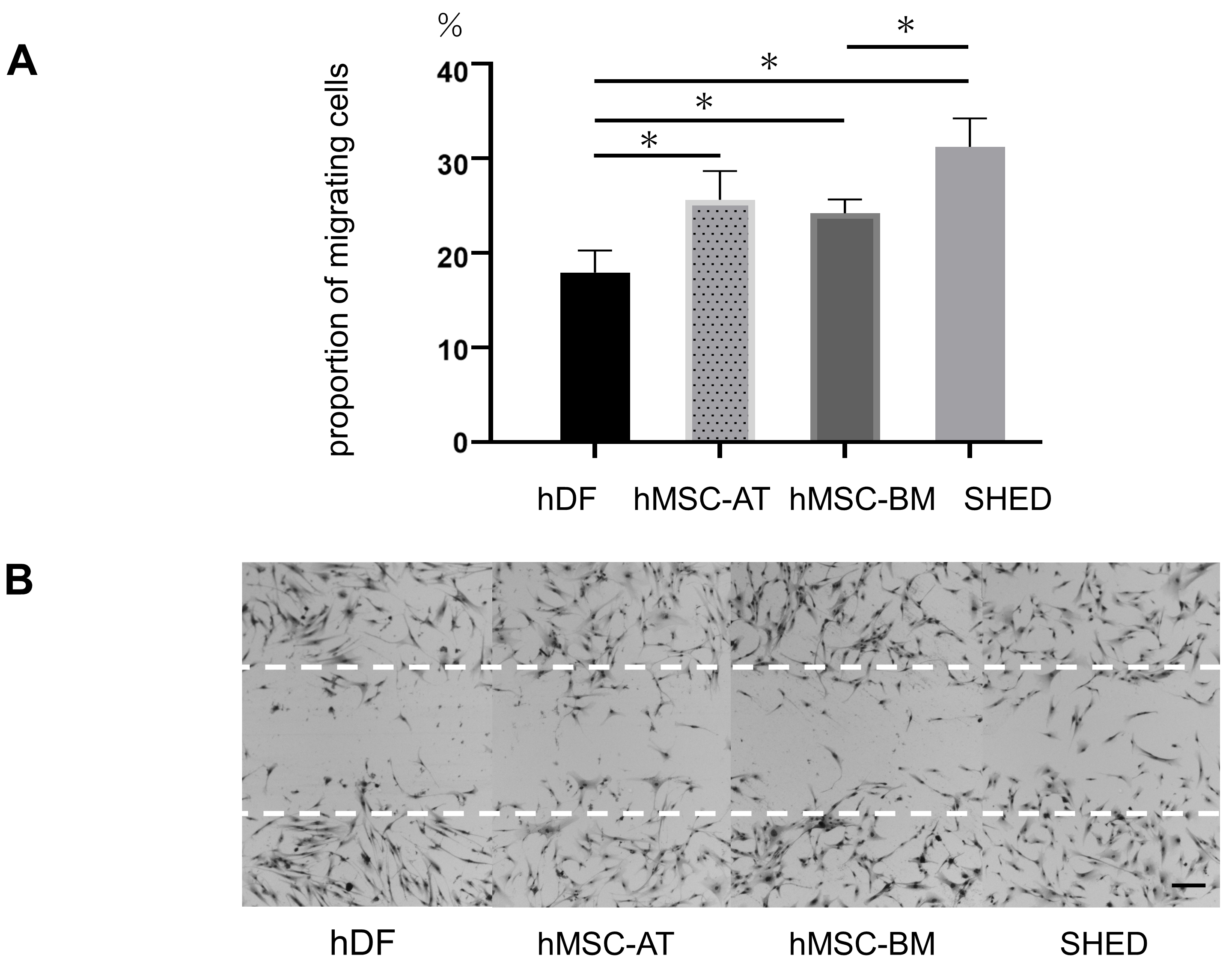
Verifying the mobility of stem cells from different sources.
(A) Quantitative data of cell motility. All stem cells showed some evidence of
increase motility, including SHED, hMSC-AT, and hMSC-BM, which were all
significantly more mobile than hDF. Data are presented as mean
The migration ability of SHED towards the CM of malignant glioma cells was
compared with those of hMSC-AT and hMSC-BM in transwell assays. hDF were used as
a control. The numbers of migrating cells towards the CM of malignant glioma were
calculated as follows: SHED, U87: 221.7
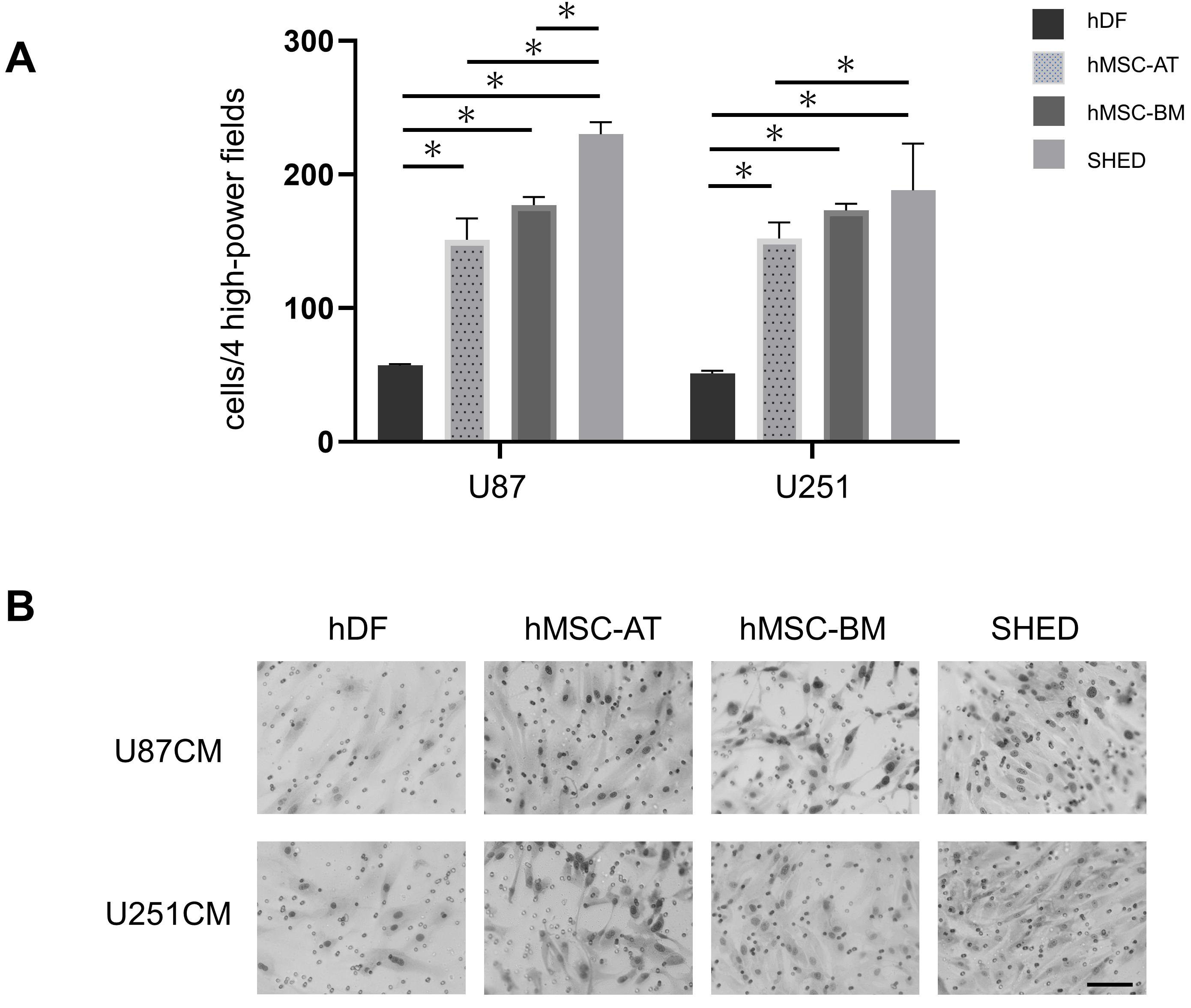
In vitro migration to glioma conditioned medium.
Induced migration of mesenchymal stem cells (SHED, hMSC-AT, and hMSC-BM) and hDF
(control) in 24-well Matrigel chambers by conditioned medium (CM) from U87 and
U251 human glioma cells. After 24 h, the number of cells that had migrated was
observed under a microscope. (A) The total number of migrated cells in four high
power fields. The number of migrated mesenchymal stem cells was significantly
increased compared with that in hDF. Data represent the mean number of migrated
cells
We also evaluated the migration of SHED to four specific growth factors with transwell assays. For all four growth factors, the number of migrating SHED cells increased in a concentration-dependent manner (Fig. 3).
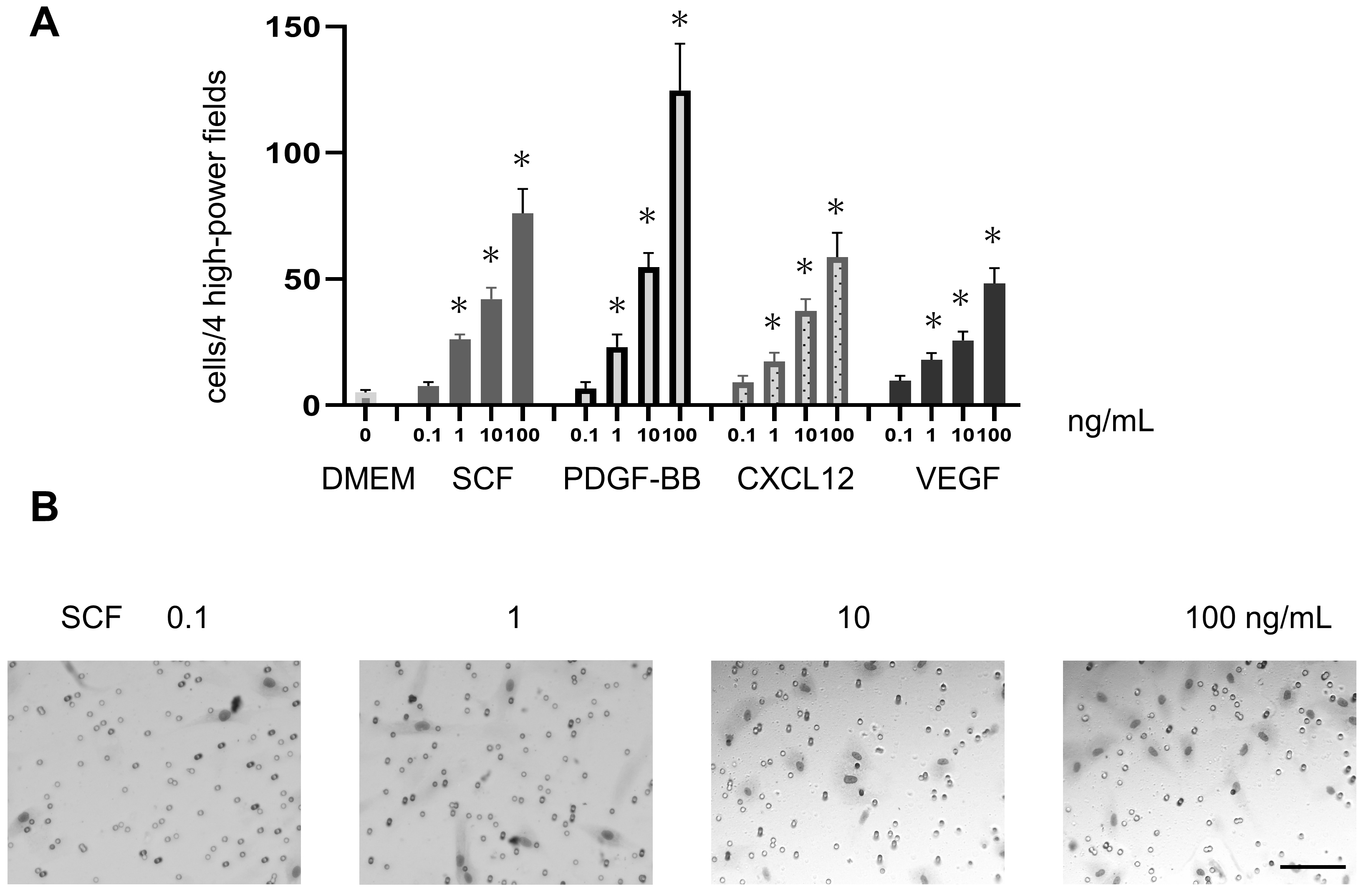
In vitro migration of SHED towards growth factors
secreted by gliomas. (A) Migration of SHED towards four specific growth factors
(SCF, PDGF-BB, CXCL12, and VEGF) in a 24-well Matrigel chambers. The migration of
SHED was significantly increased following stimulation with each growth factor in
a concentration-dependent manner (concentrations of 0.1, 1, 10, and 100 ng/mL
compared with that with Dulbecco’s modified Eagle’s medium [DMEM]). Data
represent the mean number of migrated cells
The number of migrating SHED towards CM of both U87 and U251 cells (malignant glioma cell lines) was decreased in a concentration-dependent manner by adding growth factor-neutralizing antibodies. This suggested that SHED might be attracted to these growth factors secreted by malignant gliomas (Fig. 4).
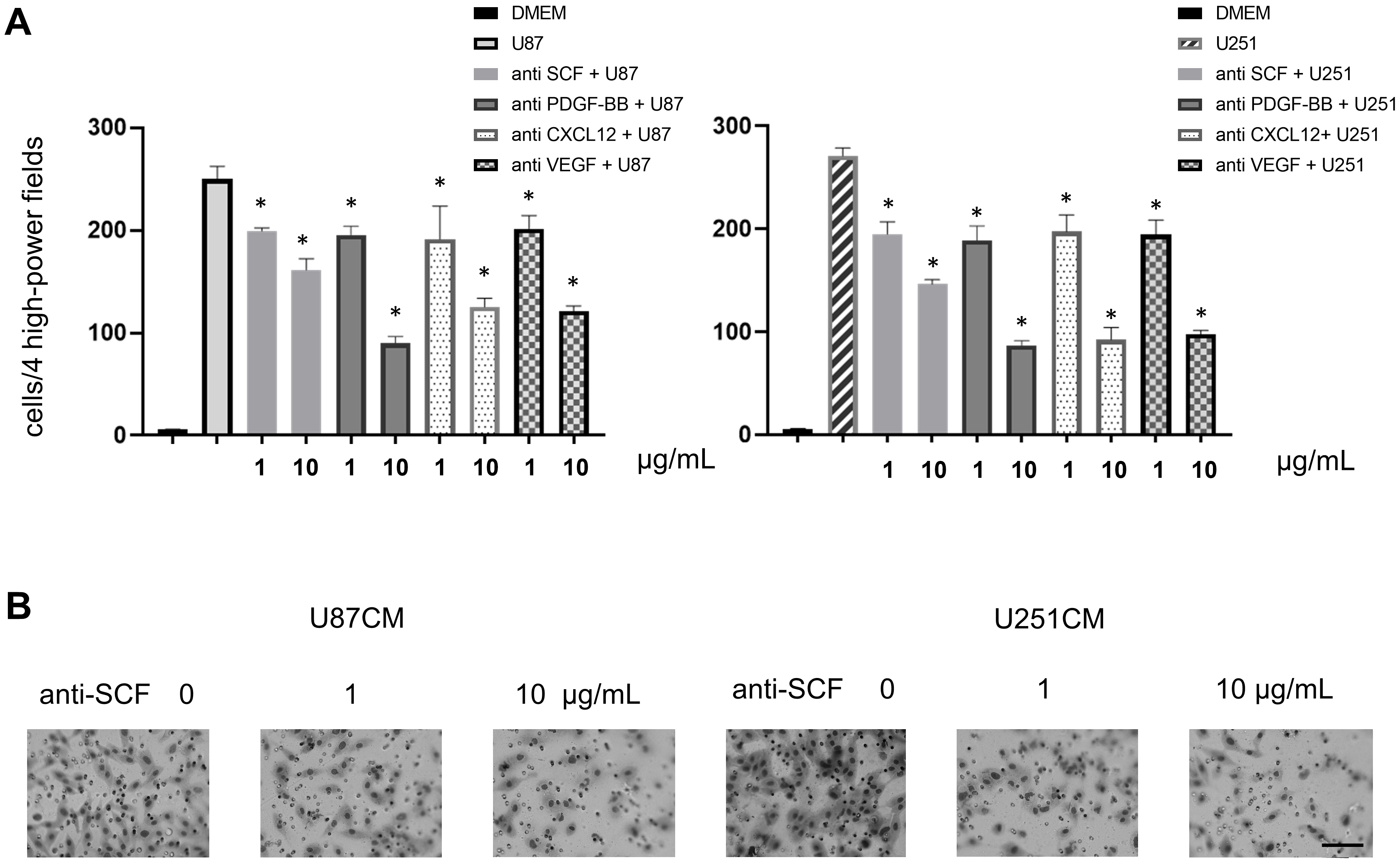
SHED migration was inhibited by neutralizing antibodies against
four growth factors. (A) The migration of SHED towards conditioned media (CM) of
U87 and U251 cells was evaluated with inhibitory neutralizing monoclonal
antibodies against the indicated growth factors at concentrations of 1 and 10
We evaluated the migration of SHED from the contralateral hemisphere to malignant glioma in the brains of nude mice. Brain sections harvested 7 d after SHED implantation showed that SHED accumulated around the tumor in the contralateral hemisphere of the brain (Fig. 5A). In contrast, hDF implanted as a control did not migrate to U87 tumors. Additionally, SHED did not migrate around human astrocytes transplanted as a control for U87 cells (Supplementary Data 2).
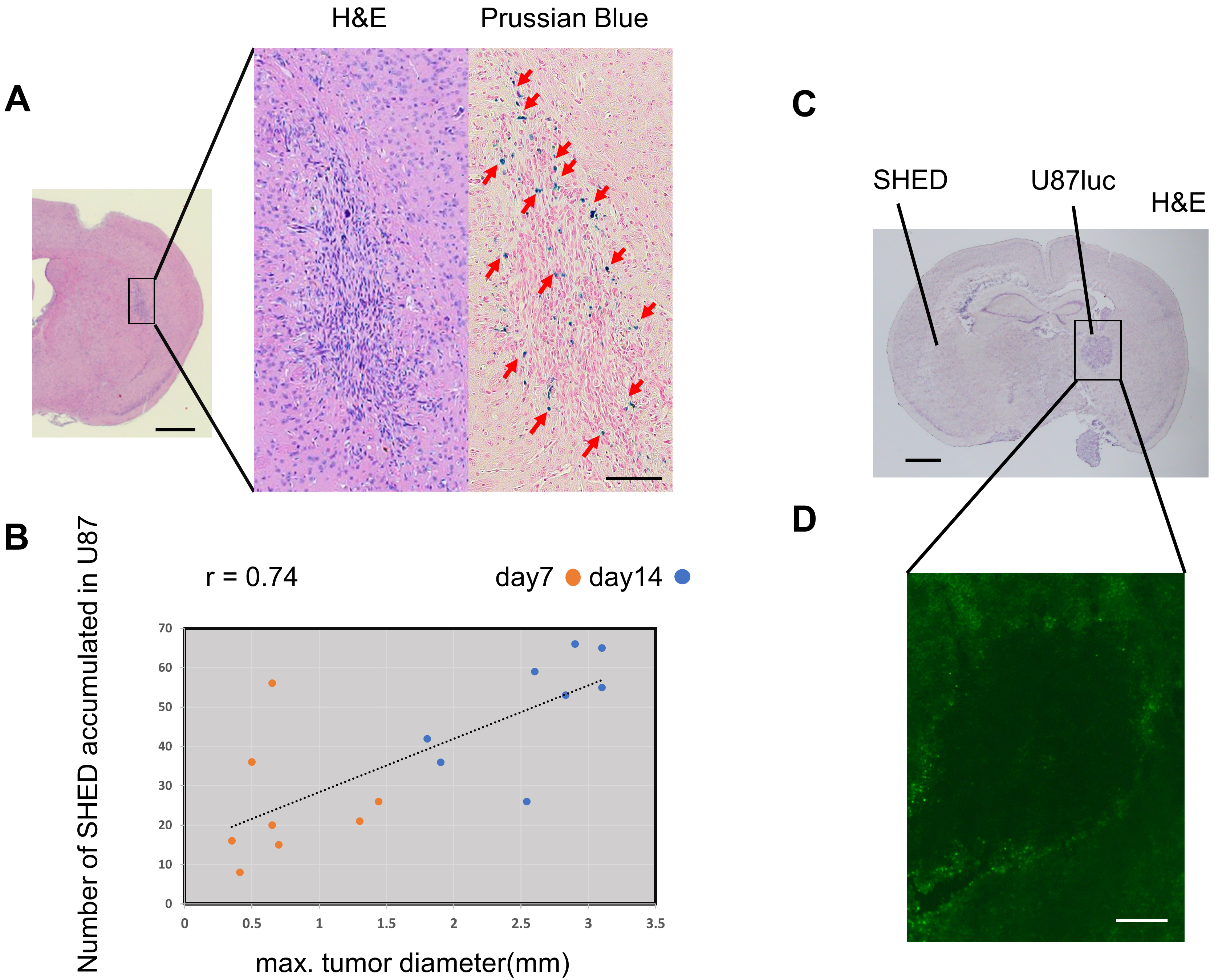
Tropism of SHED to glioma cells in vivo. (A)
SPIO-labeled SHED were implanted into the left hemisphere of BALB/c nude mice (n
= 8), and the number of SHED that accumulated around along the longest diameter
of a section of U87-luc tumors previously implanted in the right hemisphere were
counted. Representative Prussian Blue-stained images, in which SPIO-labeled SHED
are blue. Hematoxylin and eosin (H&E) staining; scale bar: 1 mm. Prussian
Blue-staining; scale bar: 100
By labeling SHED with SPIO, we could count the number of cells that had
accumulated around the tumor. The number of SHED that accumulated was positively
correlated with tumor size (r = 0.74, p
SHED were implanted into the brains of nude mice, which were evaluated for tumor formation. The mice implanted with SHED showed the same trend of weight gain as the control group, and there were no obvious changes in their health conditions. SHED implanted in the mouse brain were confirmed to be present in the mouse brain after 7 d (Fig. 6A) and 150 d (Fig. 6B) with human mitochondrial antibodies. After 150 d of SHED transplantation, there was no evidence of SHED forming tumors in brain sections from the mice (Fig. 6B).
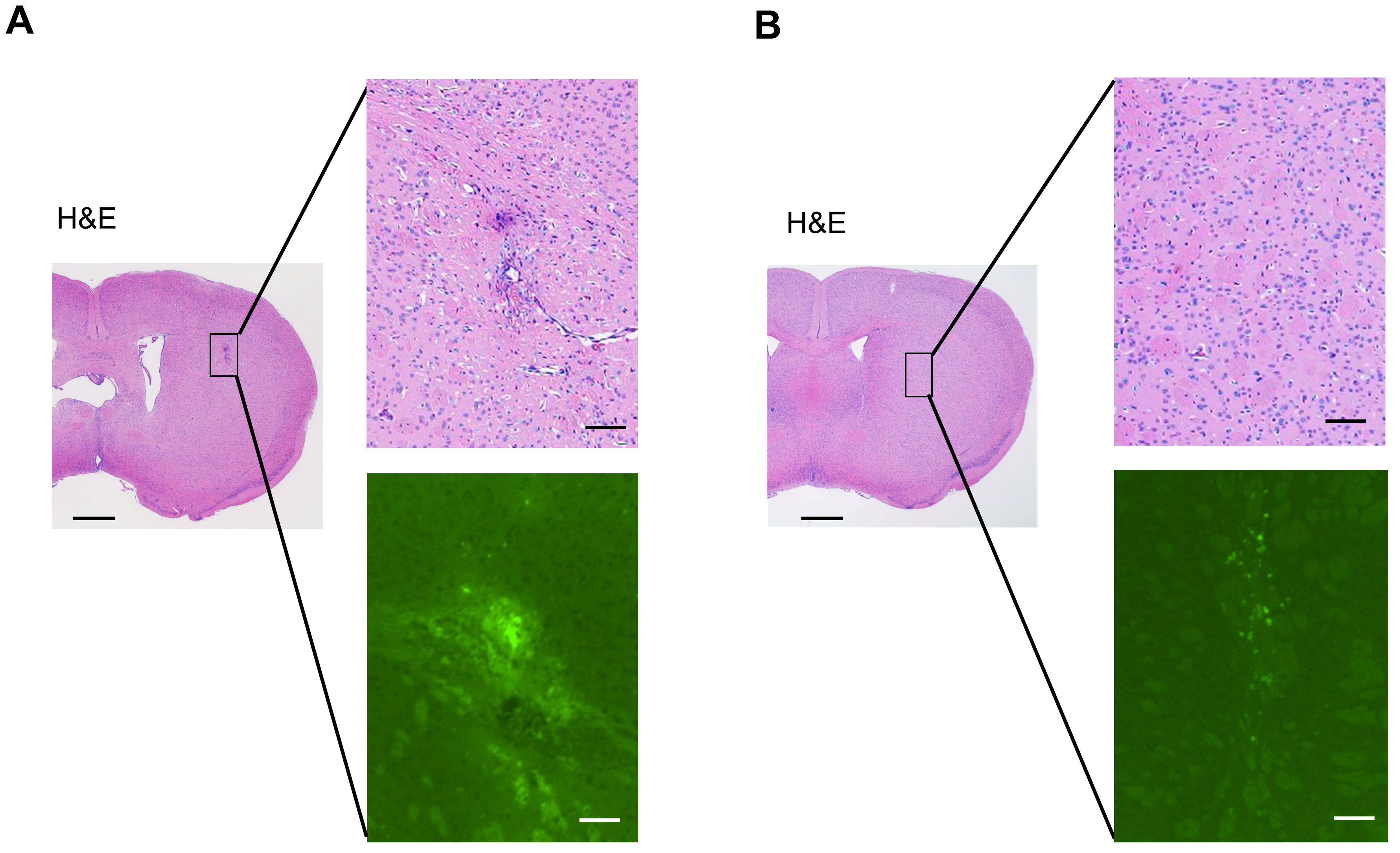
The situation of tumor formation after SHED implantation. Brain
sections of mice at 7 d (A) and 150 d (B) after SHED implantation are presented.
Upper column is hematoxylin and eosin (H&E) staining, and lower is
immunostaining for anti-human mitochondrial antibodies. As shown in the H&E
staining, SHED showed no malignant transformation or engraftment in the mouse
brain. As shown by the immunostaining, SHED were still present in the mouse brain
150 d after transplantation. H&E staining; scale bar: 1 mm. Immunostaining for
anti-human mitochondrial antibodies; scale bars: 100
In this study, we verified the migration ability of SHED toward glioma cells in vitro and in vivo and produced safety data for using SHED. In vitro transwell assays showed that SHED migrated significantly more to the CM of malignant glioma cells than hMSC-AT or hMSC-BM and were attracted to four specific growth factors secreted by malignant glioma. In vivo, SHED migrated from the contralateral hemisphere to the corpus callosum and accumulated around the tumor site in the mouse brain. Finally, after implanting SHED, there was no evidence of tumor formation in the mouse brain, even after 150 d. To the best of our knowledge, this is the first report to verify the tumor-targeting potential of SHED, and these data suggest that SHED could be used as a vehicle for future gene therapy against glioma.
MSC are self-renewing, pluripotent and have been identified in many human tissues, including bone marrow, fat, cord blood, amniotic fluid, and dental pulp. In recent studies, MSC have received particular attention in regenerative medicine and stem cell therapy [27]. Among the MSC, DPSC have recently been used for treatment as multipotent stem cells because they have neuroprotective and anti-inflammatory effects. DPSC are also being applied to treat trauma and stroke [34]. DPSC are also known to have high migratory capacity, and there have been reports on factors affecting DPSC migration [35]. SHED are a type of DPSC that were recently identified as an ideal cell type for regenerative medicine [36]. SHED are known to have no risk of tumorigenesis, high proliferative potential, multipotency, and a low risk of immunorejection [37, 38, 39]. SHED have also been investigated for treating neonatal hypoxic brain injury [40], ulcerative colitis, spinal cord injury, and Parkinson’s disease [41]. SHED can be managed by cryopreservation [36], and SHED banks have been established for autologous and allogeneic cell therapies [38, 39, 42].
Glioma microenvironment plays an essential role in controlling tumor growth and spreading. This microenvironment is made up of different cell types: tissue-resident cells, myeloid cells, bone marrow-derived cells, immune cells, endothelial cells, pericytes, and fibroblasts. In addition, glioma cells produce cytokines, chemokines, and growth factors to form tumor microenvironment [43, 44, 45].
There have been many reports on the migration of MSC to gliomas, and it is likely that various factors secreted by gliomas affect the migration of MSC to this disease [17, 46]. In this study, SHED showed significant migratory ability to the CM of two human glioma cell lines and to four specific growth factors secreted by glioma. In blocking experiments performed on CM of human glioma cells with inhibitory monoclonal antibodies against the four growth factors, SHED migration to the CM of U87 and U251 cells was significantly inhibited by all four neutralizing antibodies, indicating that these four specific growth factors significantly affect the migration of SHED.
Previously, in vitro migration assays have reported that NSC and MSC migrate to specific growth factors, particularly SCF, PDGF-BB [16], CXCL12, and VEGF [47, 48]. We also found that iPS cells recognized a variety of factors secreted by malignant glioma cells as triggers for migration [15]. Hence, SHED could likely serve as carriers for gene therapy treatments for malignant gliomas if they are genetically modified to express therapeutic transgenes.
Regarding future studies, we are in the process of verifying the receptors for the four specific growth factors (SCF, PDGF-BB, CXCL12, and VEGF), and comparing the levels of their expression between SHED and other MSCs. Additionally, we also need to confirm other factors for migration into glioma.
In vivo, SHED accumulated around tumors implanted in the contralateral hemisphere. Moreover, the number of SHED that migrated to the tumor was increased with tumor size.
NSC and MSC are excellent carriers for gene transfer into gliomas, and thus could be used to transport localized gene therapy to residual tumor cells after surgical removal, and several studies have shown that this is true for NSC, MSC, iPS cells, and Muse cells [7, 8, 9, 15]. In rodent experiments, intratumoral injection of NSC or Muse cells expressing the TK gene followed by systemic GCV administration has shown therapeutic potential. However, ethical and practical issues with transporter collection, as well as the safety and stability of the vectors remain problems that need to be resolved. TK-transduced bone marrow cells or MSC-BM also demonstrate the bystander effect [7]; however, they also contain miscellaneous factors and lack stability [49].
Murakami et al. [50] reported the self-migrating ability of MSC harvested from the same individual dog and found that DPSC showed higher migratory ability than MSC-AT and MSC-BM. Both SHED and DPSC are derived from neural crest cells that migrate throughout the body during development to form tissues [51]. Neural crest cells have been found to have excellent migratory ability [52]. Therefore, we consider that SHED and DPSC derived from neural crest cells show higher migratory ability than MSC-AT and MSC-BM derived from mesoderm. In a study comparing SHED and DPSC, Wang et al. [53] found no significant difference in the migratory capacity of SHED and DPSC. Similarly, the age of the cell donor may affect the migration of the harvested stem cells.
SHED can be collected from deciduous teeth; hence, they have no invasiveness to the human body and no ethical issues are involved in their collection. Therefore, SHED are likely to be more ideal in terms of collection. Furthermore, dental pulp containing SHED are protected by hard teeth, and their DNA is not easily damaged; thus it remains stable [54]. SHED are therefore a potential practical candidate for a stem cell-based suicide gene therapy for glioma. With respect to the effects of SHED on the normal brain, histological sections of mouse brains 150 d after SHED implantation showed no tumorigenic tissue or remnant SHED, indicating that the safety of SHED transplantation.
In this study, we confirmed that SHED is a potential candidate carrier to deliver genes to glioma cells for gene therapy. However, there are several limitations to our study. We evaluated only four specific growth factors. The study used a nude mice model, which lacks the effect on the immune system. Furthermore, we did not investigate the migratory behavior of SHED in detail. In the future, the following issues should be further investigated: the relationship between the migratory ability of SHED and other triggering factors and receptors; the immune system and inflammation; migration pathways; and the time required for migration.
We confirmed the migratory ability of SHED for malignant glioma cells. Our findings provide experimental evidence that SHED could be a potential therapeutic vehicle to deliver genes or oncolytic viruses, which would be novel treatment approaches for malignant gliomas. These data require further verification in future clinical investigations.
CM, conditioned media; CXCL12, C-X-C Motif Chemokine Ligand 12; DMEM, Dulbecco’s Modified Eagle’s medium; DPSC, Dental pulp stem cells; FBS, fetal bovine serum; GCV, ganciclovir; H&E, Hematoxylin and Eosin; hDF, human dermal fibroblast; hMSC-AT, human MSC from adipose tissue; hMSC-BM, human MSC from bone marrow; HSV, herpes simplex virus; iPS, induced pluripotent stem; MSC, mesenchymal stem cell; Muse, multilineage-differentiating stress-enduring; NSC, neural stem cell; PBS, phosphate-buffered saline; PDGF-BB, platelet-derived growth factor BB; SCF, stem cell factor; SHED, stem cells from human exfoliated deciduous teeth; SPIO, superparamagnetic iron oxide; TK, thymidine kinase; VEGF, vascular endothelial growth factor.
Conception and design—TYamam, HN, KK. Development of methodology—TYamam, HN, KK. Acquisition of data (provided animals, acquired and managed patients, provided facilities, etc.)—SK, TO, MH. Analysis and interpretation of data (e.g., statistical analysis, biostatistics, computational analysis)—TYamas, TA. Writing, review, and/or revision of the manuscript—TYamam, SK, TO, MH, TA, TYamas, TS, HN, KK. Administrative, technical, or material support (i.e., reporting or organizing data, constructing databases)—TYamam, SK, TO, MH, YM. Study supervision—HN, KK.
This study was performed in accordance with guidelines approved by the Animal Care Committee of the Hamamatsu University School of Medicine Animal Care Facility (approval number 2020089).
Part of this work was performed at the Advanced Research Facilities & Services (ARFS), Hamamatsu University School of Medicine. We thank James P. Mahaffey, from Edanz (https://jp.edanz.com/ac) for editing a draft of this manuscript.
This work was supported by HUSM (Hamamatsu University School of Medicine) Grant-in-Aid. This study was supported by Japan Society for the Promotion of Science (JSPS) KAKENHI Grant Numbers JP20K09325 (K.K.), JP19K09523 (T.S.).
Kidswell Bio Corporation (formerly, Gene Techno Science Co., Ltd., Tokyo, Japan) generously provided SHED, and hMSC-AT cell as experimental materials. YM possesses stock options of Kidswell Bio Corporation. TA is serving as one of the Editorial Board members and the guest editor for the special issue titled Exploration of mechanisms in cortical plasticity of this journal. We declare that TA had no involvement in the peer review of this article and has no access to information regarding its peer review. Full responsibility for the editorial process for this article was delegated to RF. The authors have no other relevant affiliations or financial involvement with any organization or entity with a financial interest in or financial conflict with the subject matter or materials discussed in the manuscript apart from those disclosed.