†These authors contributed equally.
Academic Editor: Rafael Franco
Decreased upper-extremity/visuomotor abilities are frequently encountered in healthy aging. However, few studies have assessed hand movements in the prodromal stage of dementia. The evaluation of visuomotor skills in patients with Mild Cognitive Impairment (PwMCI) may have non-negligible clinical relevance both in diagnostic and prognostic terms, given the strong relationships with executive functioning and functional autonomies. In the present review paper, these issues will be disclosed by describing general pathophysiological and neuropsychological mechanisms responsible for visuomotor deficits, and by reporting the available experimental results on differences in visuomotor functioning between PwMCI, healthy controls and/or patients with dementia. Moreover, the relationships binding visuomotor and executive domains to functional autonomies will be then addressed. Finally, we will propose insights for future research.
According to the World Health Organization (WHO), almost 10 million people are diagnosed with dementia each year. Nowadays, dementia affects about 50 million people worldwide. This figure is expected to increase to 82 million in 2030 and 152 million by 2050 [1]. Predictably, dementia imposes an extreme economic cost that is globally estimated to be around 818 billion dollars per year; therefore, it represents a significant barrier to both social and economic development [1, 2]. Hence there is a need for special attention on prevention and management of dementia risk factors including age-related psychophysiological decline, unhealthy lifestyle habits [3, 4], environmental factors [5], cardiocerebrovascular diseases [6, 7], sleep disturbances [8, 9], anxiety [10, 11], and apathetic symptoms [12, 13], particularly in patients suffering from Mild Cognitive Impairment (MCI) [14, 15, 16, 17, 18].
MCI is widely considered a transitional or boundary stage between normal aging and dementia [14]. MCI prevalence increases with age, from 6.7% in individuals aged 60–64 years to 25.2% in individuals aged 80–84 years [18]. Similarly, the estimates of MCI incidence range from 22.5% in individuals aged 75–79 years to 60.1% in individuals aged 85 years and older [19]. Overall, the cumulative incidence of conversion towards dementia is about 15% in patients with MCI (PwMCI) aged 65 years and over [17, 18]. Some PwMCI may remain stable or reverse to normal cognition; however, these individuals are still at a higher risk of progression back to MCI or conversion to dementia than individuals who have never received a diagnosis of MCI [18].
Based on the clinical algorithm proposed by Petersen [14, 15, 16], the diagnosis of MCI should be carried out in the presence of subjective cognitive complaints—referred by the patient and confirmed by an informant (e.g., a relative or the General Practitioner)—associated with cognitive deficit(s) formally detected by neuropsychological testing. Furthermore, PwMCI should not exhibit impairment in functional autonomies (i.e., activities of daily living, ADL) nor signs of overt dementia. According to the cognitive profile, four syndromic phenotypes have been outlined: the amnestic MCI–single domain (aMCI) variant, the amnestic MCI–multiple domain (aMCI-md) variant, the non-amnestic MCI–single domain (naMCI) variant, and the non-amnestic MCI–multiple domain (naMCI-md) variant. The diagnosis of aMCI is postulated in the presence of a selective memory deficit. Whether memory deficits are accompanied by an impairment in at least one other cognitive domain (e.g., language, executive functions, visuospatial skills), the diagnosis of aMCI-md is instead justified. Diagnosis of naMCI is advanced when a single non-memory domain is impaired, whereas naMCI-md refers to a clinical picture characterized by impairment in multiple non-memory domains [16].
Petersen’s diagnostic criteria, however, have some limits. For instance, several studies have demonstrated that PwMCI can show poor or no awareness of their cognitive deficits (i.e., anosognosia) and therefore no cognitive complaints [20, 21, 22]. Moreover, awareness of one’s deficits is, per se, a neuropsychological domain of clinical interest; thus, PwMCI showing both memory impairments and anosognosia for memory deficits should be reclassified from aMCI to aMCI-md. In addition, some evidence has suggested that MCI is often accompanied by functional decline in both basic (BADL, e.g., feeding, dressing, bathing, toileting, grooming) [23] and instrumental activities of daily living (IADL, e.g., managing finances and medications, shopping, cooking, using the phone, doing housework, driving, using public transportation, using everyday technology) [23, 24, 25, 26]. Accordingly, the National Institute on Aging and the Alzheimer’s Association developed new criteria—mostly ignored—for MCI diagnosis. The revised criteria operationalize the concept of “preservation of independence in functional activities” in a less conservative fashion, suggesting that PwMCI typically have mild problems in performing daily activities such as paying bills, preparing a meal, or shopping. Particularly, these patients would take more time, be less efficient, and make more mistakes when performing such activities than in the premorbid stage [27]. Interestingly, in a cross-sectional study including a large sample of patients with Alzheimer’s disease (PwAD) across different stages (n = 6209), 99.8% of patients diagnosed with very mild AD and 92.7% of patients diagnosed with mild AD according to the traditional criteria could be reclassified as having MCI based on the revised criteria [28].
The integrity of both lower- and upper-extremity motor functions is needed to perform ADL. Deficits in lower-extremity functioning (e.g., gait speed, balance, lower-limb muscle strength) have been described in PwMCI [29, 30, 31]. These deficits were found to be associated with difficulties in walking, driving, using public transports, or housekeeping, and are well-known risk factors for adverse events such as falls or institutionalization [30]. Deficits in upper-extremity functioning (i.e., visuomotor deficits emerging when performing unimanual/bimanual eye-hand movements) have also been detected in PwMCI (e.g., [29, 32, 33, 34, 35, 36, 37]). Visuomotor deficits appear to predict difficulties in eating, dressing, cooking, writing, doing household chores, and driving [38, 39, 40], thus representing potential risk factors for institutionalization and poor quality of life in the elderly [41].
Historically, visuomotor abilities in MCI received little attention in terms of diagnostic/prognostic significance, probably since apraxia (deficits in motor planning), ataxia (deficits in coordinating movements), and severe neurological phenomena have been traditionally classified as clinical signs of an intermediate/advanced stage of dementia. However, available data seem to suggest that visuomotor deficits should no longer be expected to manifest only in late-stage disease, thus deserving “clinical dignity” in MCI management.
Performing everyday activities requires not only intact visuomotor functions, but also efficient cognitive functioning. In particular, executive functions, such as working memory, set-shifting, and inhibitory control, are likely the higher-level cognitive processes most involved in planning, monitoring, updating, and finalization of complex behavioral patterns [24, 42], such as those involved in ADL. Indeed, a significant association between executive functioning and ADL in PwMCI has been reported in different neuropsychological investigations [24], although some concerns remain on whether there are specific executive subdomains more involved in ADL than others.
If one takes for granted that visuomotor and executive domains are both related to ADL, it is also true that these domains are related to each other. In MCI, the existence of such a relationship has been demonstrated in some correlational studies [43, 44], as well as through the use of experimental paradigms requiring the execution of hand movements under increasing cognitive load [45]. These findings are in line with anatomical-functional data suggesting that visuomotor skills and executive functions are both supported by neural activity in the frontal territories [40]. The relationship binding visuomotor and executive skills to functional autonomies is unclear and needs to be further explored.
In this vein, the present review aims at (a) providing a synthesis of the pathophysiological and neuropsychological mechanisms related to deficits in performing hand movements, (b) analyzing the results of cross-sectional studies comparing hand movements performance of PwMCI with that of control participants and/or patients with dementia (PwD), and (c) discussing the interrelation between visuomotor and executive abilities in connection with functional autonomies in MCI. Note that MCI in Parkinson’s disease (PD-MCI) is diagnosed when the cognitive decline occurs within the context of PD [46]. This is a well-known motor syndrome characterized by some cardinal manifestations such as bradykinesia, rigidity, and tremor [47]. Given the centrality of motor symptoms in patients with PD-MCI, an in-depth discussion of this MCI phenotype is beyond the scope of this review.
The posterior parietal cortex (PPC) is an associative cortical region involved in processing and combining inputs from the motor and visual cortices, and in integrating proprioceptive and vestibular signals. These anatomical-functional relationships make PPC the pillar of visuomotor behavior. Indeed, in synergy with occipital, anterior-parietal, and cortico-subcortical motor areas, PPC plays a pivotal role in planning and controlling hand movements [48, 49, 50, 51, 52] (see Appendix Fig. 2).
During the planning of hand movements, setup of initial action kinematic parameters, including distance to be covered, trajectory, and timing, is performed. Action planning is also responsible for the selection of the target object, the way in which the object will be grasped, and the final hand position. To fulfill these aims, a large amount of information is computed, such as the metric relationships between agent and target object, the spatial (e.g., size, shape, and orientation) and non-spatial (e.g., function, weight, fragility) features of the target object, and the environment’s properties [53, 54].
In controlling the movement, the trajectory of the hand is adjusted in flight. Since agent and target rarely stay in a static relationship to each other, spatial coordinates supporting movements are updated from moment to moment [54, 55, 56]. This happens because the effector moves towards the object, the positions of the eyes and head change continuously, and the object’s location, with respect to the observer, can change quickly and in unpredictable ways (e.g., in an attempt to catch a ball). Finally, as visual scene can contain many different objects, not all relevant to the current behavior, hand movements trajectory and velocity are also adjusted in relation to irrelevant competing distractor stimuli present in the environment [57, 58].
Both planning and control of hand movements use egocentric spatial representations generated within the frontoparietal network, in which the occipitoparietal/dorsal pathway plays a crucial role [56, 59, 60, 61] (see Appendix Fig. 2). These representations, combining retinotopic and spatiotopic/body-centered coordinates, are very suitable for the needs of hand behaviors [62, 63]. Indeed, the visuomotor system exploits egocentric representations for computing the spatial position of the target object with respect to the agent’s body or body parts (e.g., eyes, head, hands) and for supporting real-time visually-guided actions in the reaching space [64, 65, 66, 67]. Some neuroimaging studies have reported a selective involvement of the precuneus, in the medial PPC, during planning and execution of visually-guided reaching movements [68, 69, 70]. Furthermore, neuropsychological evidence has suggested that patients with occipitoparietal damage typically show marked deficits in reaching objects under visual guidance (i.e., optic ataxia) [66, 71, 72, 73, 74]. These deficits may be due to the inability to perform movements according to egocentric coordinates [55, 56].
As argued before, movement is a dynamic process in which spatial coordinates guiding the action need to be continuously updated. For this reason, egocentric information can be nothing but transient in nature. Indeed, it has been demonstrated that the imposition of a short delay (e.g., two seconds or less) between object presentation and movement execution may produce a rapid decay of egocentric information, with movements being less accurate, slower, and trajectories more curvilinear than observed during actions towards visible targets [55, 64, 75, 76].
In connection with the principles regulating action planning and movement control, human hand movements are organized, under normal conditions, into two consecutive phases [77, 78]. The first phase, governed by feedforward mechanisms, covers about 90% of the movement, i.e., planning and initial motion control. It is based on preexisting internal models/representations and thus requires intact memory functions. These models encapsulate information about the sensory consequences of movements such as accuracy, direction, and speed. Almost immediately after the movement initiation, limb afference and efference—concerning, for instance, movement’s speed—are compared to the expectancies associated with internal models. In case of discrepancy between the expected and observed movement’s consequences, corrections are made for aligning the ongoing motion kinematics with the predicted ones [77, 78]. In sum, people perform hand movements mainly on the basis of their predictions. For instance, according to the Fitts’ Law [79], when required to execute a pointing movement towards a small/far target, people are slower as compared to when they reach a large/near target. This might happen because people expect, based on prior experience, that a small/far object is more difficult to reach accurately than a large/close one. Therefore, people unconsciously operate a “speed-accuracy trade-off”, i.e., they tend to sacrifice speed in order to maintain acceptable levels of accuracy.
This complex no-feedback-based adjusting process is mainly supported by the parieto-fronto-cerebellar network (see Appendix Fig. 2). The cerebellum receives a copy of the motor command from the primary motor cortex and concurrently sensory inputs from the parietal cortex. Hence, by comparing these two information sources, the cerebellum predicts the sensory consequences of the motor command and prepares the musculoskeletal system to execute the movement successfully. During the movement execution, expected sensory consequences are compared with incoming information. If a positive match is detected, the same pattern is repeated in the next movement. Conversely, in the absence of a positive match, an alert signal is sent to the frontal cortex and spinal cord for recalibration of the motor plan in line with expectations [80, 81, 82]. The detection of a discrepancy between expected and actual feedbacks has a considerable evolutionary relevance, as it may be used to correct future predictions and allow more effective interaction with the environment [80, 81].
The second phase covers about the last 10% of the movement and is responsible for the implementation of in-flight corrections by visual and proprioceptive feedbacks during the deceleration phase, i.e., when the hand is close to the target [77, 78]. More precisely, visual and proprioceptive information about limb position is compared to visual information about the target position, thus allowing further adjustments of the limb trajectory and/or movement speed in order to accurately reach the target [78, 83]. Taking the above example on the Fitts’ Law, reaching a small/far object may take longer than reaching a large/near object even because the larger distance and the smaller size probably entail a more extensive intervention of feedback-based compensation mechanisms. These mechanisms seem to be mainly regulated by posterior parietal territories, including occipitoparietal/dorsal stream [50, 84], and take advantage of intact egocentric spatial representations [83].
Overall, hand movements efficiency appears to depend primarily on feedforward/predictive mechanisms and only modestly on perceptual feedbacks. However, these two processes are not independent but rather interact mutually with each other. One might expect that hand movements are not affected by impairment in feedforward mechanisms if feedback-based compensatory processes are spared. This is not the case. For example, poorer hand motor performance in the elderly, as compared with young adults, has been associated with morphological and functional deterioration of the parieto-fronto-cerebellar network (i.e., the neural substrate of feedforward mechanisms) resulting from normal aging [85]. Similarly, patients with brain damage involving the same network have been found to be slower in performing reaching movements than control participants [82, 86, 87]. These observations underpin a selective impairment of feedforward mechanisms leading to internal models’ degradation, which results in a massive use of visual and proprioceptive feedbacks (e.g., hand vs. target position) that, by nature, are slower and less accurate than internal models for controlling and correcting hand movements [82, 87]. In a specular way, some studies on healthy subjects [88] and PwAD [86, 87] have suggested that viewing the hand prior to the movement onset (i.e., visual feedback) is needed to update internal models of the starting position, to compute the distance between starting and final positions, to plan movement direction, and to reduce movement variability and directional bias. It is therefore clear that the integrity of both feedforward and feedback-based mechanisms is necessary for planning and controlling hand movements: feedback-driven adjustments make use of information stored within the internal models to ensure speed and precision of movements; however, although internal models account for the complex geometry of the eye-head-shoulder system, their contribution to the efficiency of the eye-hand motor behavior is significantly affected by the gaze direction [89]. In sum, the human brain is able to transform a stimulus encoded at the retina level into a motor command for controlling hand movements and, in order to do that, it integrates feedforward and feedback-based transformations into one functional perceptual-motor construct.
Healthy aging is associated with decreased hand motor function, as indexed by difficulties in controlling and modulating force [90], reduced coordination of finger and wrist movements [91], poor coordination of bimanual [92] and eye-hand movements [93], and increased variability [91] or slowing [92] of hand movements. Possible explanations for the age-related decline of hand motor skills include reduced physical activity and sedentary lifestyle, loss of muscle mass [85, 92], higher susceptibility to distractor interference [57], atrophy and/or abnormal activity of frontal and parietal cortices, degeneration of the cerebellum, motoneuron reorganization, impairment of the dopaminergic system [85, 92], and reduced interhemispheric connectivity [94]. All these factors are involved in the etiology of visuomotor integration deficits. For instance, the loss of muscle mass may determine dysfunction of proprioceptive receptors resulting in the weakening of proprioceptive-based corrections; cerebellar structural abnormalities could lead to the degradation of internal models for feedforward control; the deviation of the gaze towards a distracting stimulus might de-synchronize the geometric organization of internal models by producing an “overestimation” of the distracting portion of the visual field, with a negative impact on the movement’s trajectory and accuracy.
Although visuomotor deficits are frequently encountered in the healthy elderly population, PwMCI often demonstrate more pronounced deficits than healthy older adults, in some cases comparable to those observed in PwD. These observations support the view of visuomotor impairment as a continuum between healthy aging and the prodromal stage of dementia. Therefore, a comprehensive and quantitative assessment of visuomotor abilities in older adults might help to discriminate between a physiological age-related motor decline and early signs of neurocognitive impairment.
To provide a synopsis of the available experimental studies comparing PwMCI with healthy controls (HCs) and/or PwD on tasks assessing visuomotor abilities, a systematic search in PubMed was performed with no year restriction by using the following string: (“mild cognitive impairment” OR “mci” OR “mild dementia” OR “early stage dementia”) AND (“motor” OR “visuomotor” OR “movement” OR “coordination” OR “dexterity” OR “hand” OR “eye-hand”). The search ended on June 2021. Eligible studies were peer-reviewed written-in-English articles published in academic journals. Conference proceedings, letters to the editor, theses, commentaries, animals studies, single-case studies, and reviews were excluded. Furthermore, we did not consider studies assessing visuomotor abilities in PwMCI-PD because of the major motor impairment or studies using self-report measures. We complemented the search by inspection of reference lists. When more papers involved the same population, the first paper in order of publication was included. Eighteen articles were selected (see Table 1, Ref. [29, 32, 33, 34, 35, 36, 37, 43, 44, 45, 95, 96, 97, 98, 99, 100, 101, 102]).
Different methodological approaches have been used for assessing hand movements in MCI, e.g., reaching tasks [37, 95, 96], visuomotor integration tasks [45, 97], the finger-tapping task [32, 34, 43, 44, 98, 99], handwriting/graphomotor tasks [33, 35, 36, 100], and the Pegboard test [29, 32, 44, 101, 102]. The following is a brief overview of the studies included in this review.
In the experiment by Camarda et al. [95], PwAD, PwaMCI, and HCs were
asked to reach out and touch, with their right index finger, one of the six flat
LEDs placed on a table as quickly and accurately as possible. LEDs could be
placed at two distances from the starting position (15 cm and 30 cm) according to
three different spatial locations: along the midsagittal plane or laterally,
i.e., 60
While performing standard reaching tasks such as those described in Camarda’s and Yan’s studies, the participant’s gaze is directed towards the target object(s) and the hand follows the gaze direction. In other terms, the target position guides the action (i.e., standard mapping). However, there are tasks involving more complex visuomotor transformations, e.g., when gaze and action plans are dissociated or when the visual feedback is specular to the motor input (i.e., non-standard mapping). For instance, to move the cursor to the top of a computer monitor (vertical plan) one may need to move the mouse backward (radial plan). In these types of experimental paradigms, the cognitive load can be further manipulated by interposing a delay between the stimulus presentation and the movement execution, thus corrupting egocentric information. Intuitively, these tasks require more cognitive resources than a standard reaching task. Salek et al. [97] examined hand movement kinematics in PwMCI using non-standard mapping-based paradigms. Particularly, participants were asked to use their index finger for moving, via a touch screen, a cursor towards visible or remembered target locations on a display monitor under different experimental conditions. As compared with HCs, PwMCI took longer to plan and initiate movements (i.e., increased reaction times, ms) requiring visuomotor transformations of moderate complexity (two levels of dissociation between vision and action). For instance, PwMCI performed worse than HCs when asked to move the cursor by using a touch screen placed along the radial plane towards remembered target locations on a vertically-placed computer monitor. Moreover, patients showed even greater difficulties, within the same experimental apparatus, when the cursor moved in the opposite direction of the finger movement. Although the authors found no difference in movement trajectory between PwMCI and HCs, a subsequent study by Hawkins and Sergio [45] reported that PwMCI were also compromised under the movements’ trajectories profile.
Some studies found that PwMCI engaged in the finger-tapping task showed altered movement rhythm as measured by the inter-tap interval (ms) [43, 99] but no difference in movements amplitude (degrees) or velocity (degrees/s) when compared with HCs [43]. Other studies reported, instead, a decreased velocity in performing the finger-tapping task. Particularly, in Roalf et al.’s study [34], the tap counting (number of taps in 60 s) was similar for PwAD and PwMCI, with both groups showing lower tap counting than the control group. A recent investigation by Hesseberg et al. [44] reported similar findings.
When required to copy a pre-established template (e.g., the Chinese characters “Yong” or “Zheng”), PwMCI were significantly slower and less accurate in performing handwriting movements as compared with HCs [35, 36]. Movements of PwMCI were found to be slower than HCs also in spontaneous drawing tasks, e.g., to draw a tree, with similar performance observed in PwD [100]. Intriguingly, PwMCI showed no difficulties in tracing pre-drawn simple geometric figures [35].
In the study by Kluger et al. [32], PwAD demonstrated an unspecific impairment involving complex motor (e.g., Purdue Pegboard assembly test), gross motor (e.g., finger-tapping speed, steadiness, strength), and fine motor skills (i.e., Purdue Pegboard and Grooved Pegboard tests). Conversely, PwMCI showed spared gross motor, and impaired complex and fine motor skills. As for the latter, performance of PwMCI was comparable to that of PwAD [32]. In a subsequent study conducted on a larger sample of PwMCI [29], both PwMCI and PwAD performed worse than HCs on the Purdue Pegboard test, although PwMCI outperformed PwAD (see also Hesseberg et al. [44]). In the same year, Lopez et al. [101] provided converging evidence supporting the hypothesis that fine motor skills (Purdue Pegboard test) are impaired in MCI. They also demonstrated that PwnaMCI-md were more compromised than PwaMCI-md; however, both groups showed greater difficulties than PwaMCI. In line with these findings, De Paula et al. [102] reported that PwAD showed reduced fine motor dexterity than PwaMCI, whereas PwaMCI-md showed a similar degree of fine motor deterioration compared to PwAD.
Reference | Sample | Main assessment | Main outcome variable/s | Results |
Aggarwal et al. (2006) [29] | 60 AD, 198 MCI [NOS], 558 HCs | Pegboard Test | Manual Dexterity | HCs |
Camarda et al. (2007) [95] | 11 AD, 11 aMCI, 11 HCs | Reaching Tasks | Hand Movement Velocity | HCs = MCI |
Colella et al. (2021) [43] | 14 aMCI, 16 HCs | Finger-Tapping Task | Movement Rhythm | HCs |
Movement Amplitude | HCs = MCI | |||
Movement Velocity | HCs = MCI | |||
De Paula et al. (2016) [102] | 38 AD, 34 aMCI, 32 aMCI-md, 20 HCs | 9HPT | Manual Dexterity | HCs = aMCI, HCs |
Franssen et al. (1999) [98] | 101 AD, 69 MCI [NOS], 195 HCs | Sequential Finger to Thumb Tapping Task | Manual Dexterity | HCs |
Hawkins and Sergio (2014) [45] | 8 MCI [NOS], 44 HCs | Visuomotor Integration Tasks | Hand Movement Trajectory | HCs |
Hand Movement Velocity | HCs | |||
Hesseberg et al. (2020) [44] | 38 PwD, 60 MCI [NOS] | Finger-Tapping Task | Psychomotor Speed | MCI = PwD |
Grooved Pegboard Test | Manual Dexterity | MCI | ||
Huang et al. (2019) [35] | 36 AD, 43 MCI [NOS], 41 HCs | Spiral Examination | Hand Movement Velocity | HCs = MCI = AD |
Yong Examination | Hand Movement Trajectory | HCs | ||
Hand Movement Velocity | HCs | |||
Kluger et al. (1997) [32] | 25 AD, 25 MCI [NOS], 41 HCs | Finger-Tapping Task | Gross Motor Skills | HCs = MCI |
Purdue Pegboard Test | Fine Motor Skills | HCs | ||
Grooved Pegboard Test | Fine Motor Skills | HCs | ||
Purdue Pegboard Assembly Test | Complex Motor Skills | HCs | ||
Lopez et al. (2006) [101] | 10 aMCI, 22 aMCI-md, 6 naMCI-md, 374 HCs | Grooved Pegboard Test | Fine Motor Skills | HCs = aMCI, HCs |
Mitchell et al. (2020) [37] | 17 AD, 10 aMCI, 24 HCs | Reaching tasks | Hand Movement Velocity | HCs |
Roalf et al. (2018) [34] | 131 AD, 46 MCI [NOS], 62 HCs | Finger-Tapping Task | Fine Motor Skills | HCs |
Robens et al. (2019) [100] | 56 AD, 64 MCI (aMCI and aMCI-md), 67 HCs | Digital Tree Drawing Test | Hand Movement Velocity | HCs |
Salek et al. (2011) [97] | 10 MCI (aMCI, aMCI-md, and naMCI-sd), 10 HCs | Visuomotor Integration Tasks | Hand Movement Trajectory | HCs = MCI |
Hand Movement Velocity | HCs | |||
Schröter et al. (2003) [33] | 35 AD, 39 MCI [NOS], 40 HCs | Handwriting Task | Manual Dexterity | HCs |
Suzumura et al. (2018) [99] | 31 AD, 15 MCI [NOS], 48 HCs | Finger-Tapping Task | Finger Dexterity | HCs |
Yan et al. (2008) [96] | 9 AD, 9 aMCI, 10 HCs | Fitts-like Paradigm | Hand Movement Velocity | HCs |
Hand Movement Smoothness | HCs | |||
Yu et al. (2019) [36] | 22 AD, 14 aMCI, 18 HCs | Graphomotor Task | Hand Movement Accuracy | HCs |
In sum, except for a few studies finding no hand movement deficits in MCI [32, 35, 43, 95, 101, 102], the majority of experimental evidence suggests that hand movements in PwMCI are slower [35, 37, 45, 96, 97, 100], less coordinated [29, 32, 33, 34, 43, 98, 99, 101, 102], and less accurate [35, 36, 45] as compared with HCs. Interestingly, some studies have also reported a similar degree of visuomotor deterioration in both MCI and overt dementia [32, 34, 36, 44, 100, 102] (see Table 1). Therefore, this pattern of results supports the view that hand movement deficits may occur well before signs of severe motor impairment, which are typical of a more advanced stage of the disease. Moreover, part of this evidence suggests that MCI severity, according to the specific clinical subtype, might moderate the development of visuomotor deficits. Nevertheless, it is important to underline that available studies are highly heterogeneous both in terms of sample size/characteristics and assessment methods used. This heterogeneity makes it difficult to draw exact conclusions about gravity, dimensionality, and extension of hand motor deficits.
The pathophysiological mechanisms related to hand movements deficits in MCI are still unclear. Among the most convincing hypotheses are dysfunction of the frontoparietal network [29, 32, 36, 43, 95], abnormal activity of posteromedial parietal regions such as posterior cingulate cortex and precuneus [22, 37], neuropathological lesions of the pulvinar and extra-pulvinar network (e.g., superior colliculus and posterior parietal cortex) [103], periventricular white matter lesions in the frontal lobe [104], and accumulation of neurofibrillary tangles in the substantia nigra [105] (see Appendix Fig. 2 for a focus on the nigrostriatal pathway). In conjunction with these pathophysiological alterations, it has been hypothesized that visuomotor impairment in MCI is likely due to attentional/filtering or inhibition deficits [43, 103] and/or severe internal models’ degeneration leading to the selective use of feedback-based control mechanisms [37, 86, 87].
As concerns the frontoparietal network, it is well-known that it plays a pivotal role in transforming visuospatial information into intrinsic joint and muscle representations that guide the initial motor outputs of goal-directed hand movements. Interestingly, diffusion tensor imaging (DTI) studies on PwMCI showed an early disconnection between parietal and frontal cortices likely responsible for the observed visuomotor deficits [45, 106].
Within the frontoparietal network, the PPC makes a fundamental contribution in the space-time coordination of eyes and hands movements; furthermore, it transforms visual, proprioceptive, and vestibular signals into adjusting motor commands based on egocentric coordinates [95]. In support of these claims, neuroimaging evidence has suggested a striking involvement of the medial PPC in the planning and execution of visually-driven reaching movements [22]. Net of some speculations, neuroimaging studies revealing a direct/causal link between a selective parietal dysfunction and visuomotor impairments in MCI are not currently available.
The pulvinar, in synergy with the occipitoparietal cortex and the superior colliculus (see Appendix Fig. 2), supports a wide range of cognitive/motor processes (e.g., selective attention, filtering, visual scanning, eye movements, visuospatial orientation, color and form recognition) required to properly perform different visuo-spatial/-motor activities [107, 108, 109]. A recent study, using a novel MRI-based thalamic segmentation technique [110], compared PwAD, PwMCI, and HCs with respect to the volume of thalamic nuclei, including the pulvinar. Results of analysis of covariance adjusted for sex and intracranial volume showed that most of the thalamic nuclei were significantly smaller in PwMCI/PwAD than in HCs. Furthermore, the mediodorsal, medial geniculate, and pulvinar nuclei were significantly smaller in PwMCI than in HCs.
Regular patterns of frontal subependymal lesions have been associated with decreased lower-extremity mobility in PwMCI [104]. However, to the best of our knowledge, no studies have investigated the possible relationship between periventricular leukoaraiosis and upper-extremity motor function in MCI.
Hyperphosphorylated tau protein accumulation in neurofibrillary tangles within the nigrostriatal pathway has been associated with motor deficits in the elderly with and without dementia [29, 105]. In PwMCI, clusters of tau deposition have been observed, mainly in the left hemisphere, in the frontal and temporal cortices, as well as in subcortical structures including thalamus, caudate nucleus, and putamen [111]. Interestingly, it has been reported that abnormal activity in the substantia nigra may be (i) considered a robust biomarker for distinguishing MCI due to Lewy Body Dementia (LBD) from MCI due to AD [112] and (ii) useful for identifying subjects at high risk of progression towards dementia [113].
As already mentioned in the Introduction of this paper, hand movements are strongly associated with ADL. For instance, planning and control of hand movements are needed to reach and grasp an object; unimanual coordination is necessary for using cutlery to bring the food from the plate to the mouth, for tooth-brushing, or for taking medications; intact bimanual coordination is required for tying shoelaces, for buttoning/unbuttoning a shirt, or for meal preparation; fine motor dexterity is needed for sewing/mending or to properly execute handwriting movements.
The relationship between visuomotor deficits and ADL has been rarely explored in
both general and clinical populations. Although some cross-sectional [114, 115]
and longitudinal evidence [115] exist about a decreased speed in performing
ADL-related tasks in PwMCI, a few studies probed the impact of visuomotor skills
on functional autonomies. de Boer et al. [116] explored the association
between deficits of visually-guided reaching movements and IADL in patients with
cognitive impairment (i.e., PwAD, PwaMCI, patients with mixed dementia, and
individuals with cognitive complaints). In this study, participants who showed a
loss of autonomy in at least two IADL domains took more time in initiating and
executing hand movements compared with participants who reported no signs of
functional decline. The study by De Paula et al. [102] yielded, instead,
promising evidence about a selective contribution of fine motor dexterity in
explaining BADL impairments in patients with neurocognitive disorders. More
recently, Schaefer et al. [117] developed a simple timed coordination
task to test the role of hand movements performance in predicting functional
decline over a 1-year follow-up in PwaMCI. Participants used their dominant hand
to convey, through a standard plastic spoon, two raw kidney beans at a time from
a central cup to one of the three distal cups arranged at a radius of 16 cm
relative to the central cup (at –40
Executive functions are also related to ADL. They refer to a family of top-down mental processes (e.g., processing speed, selective and divided attention, set-shifting, interference and inhibitory control, planning, monitoring, working memory) involved in the execution of complex action schemes in order to adaptively cope with environmental requests in unfamiliar of conflicting contexts [42, 118]. Furthermore, executive functions regulate intact and/or compensate impaired cognitive domains [24]. Some studies investigating the neuropsychological correlates of functional autonomies in healthy elderly, PwMCI, and PwD have revealed a significant association between executive functions and the ability to carry out everyday tasks [24, 119, 120, 121, 122, 123, 124, 125]. As concerns PwMCI, here we provide a short summary of the most recent evidence.
In Hackett et al.’s study [126], semantic fluency test (generativity and cognitive flexibility), Trail Making Test-A (TMT-A; processing speed, visual search, and attention skills), Trail Making Test-B (TMT-B; set-shifting and inhibition/interference control), and Symbol Digit Modalities Test (SDMT; visual scanning, sustained attention, and psychomotor speed) were found to be correlated with the Functional Activities Questionnaire (FAQ), i.e., a well-known 10-item scale designed to assess functioning across some IADL including shopping, financial management, and cooking [127]. These results were partially replicated in Nguyen et al.’ study [125] using an IADL performance-based measure. Nguyen and coworkers reported a significant correlation with the verbal fluency and similarities test (i.e., abstract reasoning and conceptualization). Furthermore, they found that TMT-B and similarities test were the cognitive measures explaining the highest amount of variance in the IADL score according to multiple hierarchical regression analysis [125]. Using a similar regression-based procedure, García-García-Patino et al. [128] found that TMT-A score was the best predictor of IADL in PwMCI.
Wadley et al. [129] calculated a cognitive composite score obtained from the assessment of several executive domains in PwMCI and mild dementia. This score entered regression models including genetic and neuroimaging markers of AD risk. The executive score was strongly associated with functional performance in MCI and mild dementia, independently of genetic risk and neuroimaging AD biomarkers. Intriguingly, by running different latent variable path models, Kwak et al. [130] suggested that executive processes mediate the neural activity of the medial temporal lobe (i.e., hippocampus and amygdala) and thalamus that, in combination with the Default Mode Network, support IADL in older adults at different degree of neurocognitive impairment.
In an attempt to root out the link binding visuomotor and executive domains to ADL, the relationship between these two domains should be further analyzed. The close relationship between motor and executive functions is predictable based on shared neural mechanisms involving cortical and subcortical frontal regions [40, 131]. However, there are only a few studies exploring this relationship in MCI.
Some studies on healthy older adults have reported a significant association between certain executive subdomains (e.g., inhibition, processing speed, set-shifting) and movement variability [132] or finger dexterity [133, 134]. In PwaMCI, Colella et al. [43] found that fewer rhythmic movements during finger tapping were associated with lower scores on the Frontal Assessment Battery (FAB). The latter is the most widely used short screening battery to assess general executive functioning (i.e., semantic categorization, cognitive flexibility, planning and execution of sequential movements, sensitivity to interference, inhibitory control) in both clinical and experimental settings [135]. In the study by Hesseberg et al. [44], hand and executive functions were assessed in PwD and PwMCI. Hand functions included psychomotor speed as measured by the finger-tapping task and manual speed/dexterity as measured by the Grooved Pegboard test. Executive tasks included instead the TMT-A and TMT-B. The results showed that performance on the finger-rapping task was mainly associated with set-shifting and inhibition/interference control (i.e., TMT-B), while performance on the Grooved Pegboard test was mainly explained by processing speed, visual search, and attentional domains (i.e., TMT-A) [44].
Other research has investigated the relationship between visuomotor and executive domains through the cognitive load manipulation in visuomotor transformation tasks. In the study by Tippet et al. [136], PwAD and HCs were instructed to slide their index finger over a touch screen and move a cursor towards a visual target under four experimental conditions. In the “standard-mapping condition” the touchscreen was placed directly over a laptop monitor, and the cursor followed the finger movements. In the “non-standard mapping/rotated condition” (strategic control), the touchscreen was placed over the laptop monitor, but the finger moved the cursor in the opposite direction. In the “non-standard mapping/dissociated condition” (visuospatial recalibration), the touch screen controlling the cursor was placed horizontally in front of the monitor where the stimuli were presented, with finger and cursor moving in the same direction. Finally, in the “non-standard mapping/dissociated-rotated condition” (strategic control + visuospatial recalibration), the touch screen and monitor were placed on dissociated planes, and the finger and cursor moved in the opposite direction. Timing and performance errors were recorded. In all the four conditions, PwAD were slower in initiating and executing reaching movements compared with HCs, although the between-group differences were more pronounced under the highest cognitively demanding conditions, i.e., the rotated ones [136]. In a subsequent study by the same research group [45], a similar experimental procedure was used to compare the performance of healthy young and older adults with that of individuals at high AD risk (i.e., family history of dementia or MCI diagnosis). As was the case for PwAD, individuals at high AD risk demonstrated visuomotor difficulties with increasing levels of cognitive demand. In particular, individuals with a family history of dementia showed a selective impairment—in terms of movement variability, accuracy, and speed—in the most cognitively demanding condition, i.e., the non-standard mapping/dissociated-rotated condition. Conversely, PwMCI performed worse than HCs in all the non-standard mapping tasks. In line with these findings, Staal et al. [137] recently proposed a classification algorithm able to distinguish with 87% accuracy PwMCI from HCs based on kinematic parameters recorded during the execution of eye-hand movements with increasing cognitive demand.
Neuroimaging evidence has suggested that decreased performance in highly-demanding visuomotor tasks could be due to reduced functional connectivity between precuneus, anterior and posterior cingulate cortex, thalamus, primary motor cortex, and primary somatosensory cortex [138]. More specifically, lower accuracy/higher variability of hand movements seems to be associated with reduced functional connectivity between frontal and parietal cortex, as well as between temporal cortex and subcortical regions (i.e., thalamus and basal ganglia). Besides, decreased functional connectivity between frontal cortex and precuneus, as well as between frontal and temporal cortices, appears to be associated with hand movements slowness [138].
Finally, Lagun et al. [139] constructed an algorithm to improve the accuracy in detecting MCI by using the Visual Paired Comparison task (VPCT). The VPCT consists of a familiarization phase followed by a test phase. During the familiarization phase, two identical visual stimuli were presented side-by-side on a computer screen. After a short delay (e.g., 2 s), the test phase starts, and two stimuli, an old and a new one, are displayed side-by-side. Eye movements of the participants are recorded via eye-tracking. Typically, during the test phase, HCs spend 70% of the time looking at the novel stimulus, while PwMCI do not exhibit a significant preference for the new stimulus. This suggests that PwMCI are unable to retain in working memory the old stimulus, which results in an undifferentiated fixation pattern. These observations might partially explain some abnormalities reported in PwMCI when performing eye-hand movements. The algorithm employed by Lagun et al. demonstrated excellent discriminative capability, being able to differentiate PwMCI from HCs with 87% accuracy [139].
Overall, according to the above overview, executive functions and visuomotor abilities are related to each other; however, the direction of this relationship is still unclear. Then one question arises: how much do executive functions affect visuomotor skills, and how much do visuomotor skills affect the assessment of executive functions? On the one hand, it has been observed that hand movements deficits would emerge primarily when the task is highly demanding, thus consuming greater executive control resources [45, 140]. On the other hand, for instance, patients with behavioral variant frontotemporal dementia (bvFTD) commonly show executive deficits in the early stage of the disease as a result of orbitofrontal, dorsolateral and medial frontal cortices neurodegeneration; however, neurological signs of visuomotor alterations occur only with disease progression [141]. The above question assumes even more weight given that many tests assessing executive functions require a motor response. Thus, the evaluation of some executive domains, e.g., set-shifting, processing speed, inhibition, or interference control, may be affected not only by top-down cognitive mechanisms but also by manual speed and dexterity. Accordingly, future studies could further investigate such a relationship by administering executive tasks not requiring a motor response, for instance, the Stroop test [142], the phonemic/semantic alternate fluency test [143], the oral TMT [144], or the SDMT–oral version [145]. Moreover, one might consider the possibility of extracting normative data for movement-based executive tasks by taking into account not only socio-demographic variables (i.e., sex, age, and education) but also kinematic variables (e.g., manual speed, accuracy, and coordination).
In sum, hand motor and executive domains may represent independent predictors of functional autonomies in PwMCI and PwD. Since both domains appear to be related to each other, future research could test two predictive mediation models to disentangle the complex relationship binding visuomotor and executive abilities to ADL (see Fig. 1).
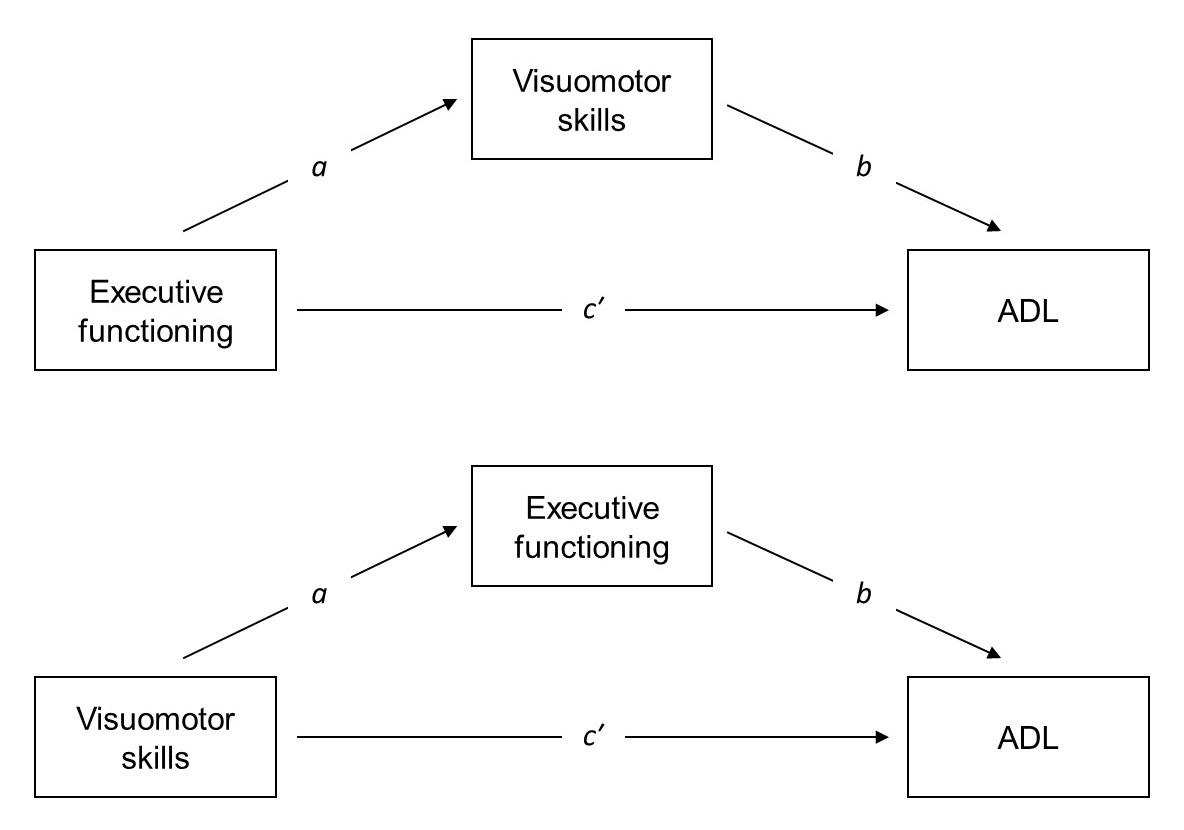
Schemes representing the two proposed mediation models for investigating the relationships between visuomotor skills, executive functions, and activities of daily living (ADL). The first model (upper figure) includes visuomotor abilities as mediator of the relationship between executive functioning and ADL. The second model (bottom figure) includes executive functioning as mediator of the relationship between visuomotor abilities and ADL. a = Effect of independent variable on the mediator. b = Effect of the mediator on the dependent variable. c′ = Direct effect.
Visuomotor tasks, unlike verbally-based cognitive tasks, are relatively unaffected by formal schooling and language abilities. Thus, they represent suitable and flexible tools for investigating the presence of mild neurocognitive deficits among older adults regardless of education levels [136, 146]. Moreover, the decline in visuomotor functioning seems to be associated with cognitive [43, 45] and functional decline [117]; this makes it a dimension of great clinical interest both in diagnostic and prognostic terms.
As pointed out in the present review, assessment of hand movements can be useful for differentiating PwMCI from HCs. However, it is unclear whether the examination of upper-extremity motor function could actually discriminate between MCI and overt dementia (see Table 1). In this vein, cross-sectional studies comparing HCs, PwMCI at different degrees of cognitive impairment, and PwD are needed to determine whether and how MCI severity modulates the onset of visuomotor disorders. Furthermore, such studies could provide additional evidence about the possibility of discriminating among different MCI clinical phenotypes through visuomotor examination and about its utility in the differential diagnosis between MCI and early/mild dementia.
It is important to underline that the available data are highly heterogeneous, especially regarding the assessment methodology, which varies from classical cognitive tests to more complex computer-based procedures. As hand movements have been mainly studied in experimental settings, there is still no consensus regarding the best clinical protocol for the evaluation of hand movements in MCI. Preference should be given to flexible, no-time-consuming, and economic tools so that these can be administered within composite neuropsychological batteries during the outpatient clinical practice. For instance, the Pegboard test may be employed for assessing manual dexterity; the finger-tapping task—which can be easily performed by means of a counting machine [44]—to measure speed and coordination of hand movements.
It seems that visuomotor deficits in PwMCI are more pronounced under high cognitively demanding conditions [45, 137]. Consequently, easy-to-use visuomotor measures permitting the manipulation of the cognitive load should be designed and complemented by robust psychometric investigations, including validation and normative studies, on large samples of older adults. Particularly, phase I psychometric trials should aim at ensuring task uniformity, as well as providing a precise number of trials varying in complexity, including a clear description of the respective cognitive load. As concerns the already available tools, the traditional finger-tapping paradigm may be integrated with a sensorimotor synchronization condition, e.g., asking the patient to synchronize her/his finger movements with a variable auditory rhythm. This variant, which might be implemented in a smartphone-based application, would provide an estimate of accuracy (i.e., how much the finger taps coincide with the rhythm) and allow for manipulation of the cognitive load (i.e., from slow-to-fast rhythms). An additional promising tool is the Visual-Motor Speed and Precision Test (VMSPT) [147]. The VMSPT is a very fast pencil-and-paper task devised to quantify the speed and precision of fine visuomotor coordination. The patient is presented with a sheet of white paper on which several little circles are printed. Proceeding from the top down, the circles decrease in size. The patient is instructed to make a cross completely inside each circle, one right after the other, as fast as possible, and without skipping any circle. One point is given for each cross placed within the circle to obtain the precision score. The total number of crosses placed within, or outside, the circle constitutes the speed score. Interestingly, this task is structured to become increasingly difficult (the circles become progressively smaller), thus challenging both speed and accuracy of movements. Validation and normative studies on large and representative samples of elderly people are required to adapt these tasks to geriatric clinical practice and to control the impact of potential threats to internal validity, such as tiredness or boredom. Notably, the experimental procedure drawn by Schaefer et al. [117] appears straightforward and relatively fast. Therefore, it might be declined in the outpatient clinical practice and restructured under variable cognitive load.
From a therapeutic perspective, seeing the relationships between hand movements, executive functions [44], and ADL [102], non-pharmacological interventions focused on upper-extremity motor exercises might improve both cognitive and functional outcomes [40, 102]. Rehabilitation programs consisting of multicomponent interventions to improve both visuomotor and executive processes might have an even greater positive impact on functional autonomies. Testing the efficacy of these types of intervention, to be preferably declined in ecological settings, could represent a relevant challenge for future research. Since PwMCI may rely almost exclusively on feedback-based control mechanisms during hand movements [37], correction of any visual problems, or the use of visual aids, can be effective in any kind of training process. Note that the relationship binding visuomotor and executive domains to ADL is still unclear. Future studies could try to isolate (or adjust) the motor component during the neuropsychological evaluation of executive functions and establish whether and how visuomotor and executive domains interact in explaining the variance of ADL performance.
Finally, as outlined in our recent paper [22], no study has explored the predictive role of visuomotor deficits on conversion from MCI towards dementia: an additional starting point for future research. It has been speculated that the assessment of visuomotor abilities may be useful to early discriminate PwMCI who are more likely to convert in dementia from those who are not [96, 97]. This speculation appears well-founded; indeed, together with visuospatial working memory and metamemory (i.e., two domains significantly and individually associated to conversion [148, 149, 150, 151, 152]), visuomotor abilities strongly depend on the activity in the posteromedial parietal cortex (PMC). PMC hypometabolism is largely considered a robust biomarker of progression to AD-like dementia [153, 154, 155]. As a consequence, we suggested that clinicians focus on this tripartite neuropsychological cluster during outpatient clinical practice using defective scores as an indirect index of PMC impairment.
In the current historical period, the early detection of individuals in the
prodromal disease stage is of paramount importance as PwMCI will be ideal
candidates for future AD treatments. To date, 126 molecules have been
synthesized, of which 28 are in phase 3 clinical trials. Among the latter, the
Aducanumab—already approved in the United States—is a human immunoglobulin G1
(IgG1) monoclonal antibody that appears to be able to successfully attack
aggregated soluble and insoluble forms of amyloid
CRI conceptualized the study. CRI, AI, and MLM analyzed the relevant literature and provided a narrative synthesis. CRI wrote the first draft of the manuscript. AI, TI and SC critically reviewed the first draft by commentaries and insightful revisions. All authors read and approved the final manuscript.
Not applicable.
Not applicable.
Dr. CR Ilardi received a Ph.D. grant from the University of Campania “Luigi Vanvitelli” (Protocol code n. 76400; date: June 5, 2019).
The authors declare no conflict of interest.
See Fig. 2 (Ref. [157, 158, 159, 160, 161]) for detail.
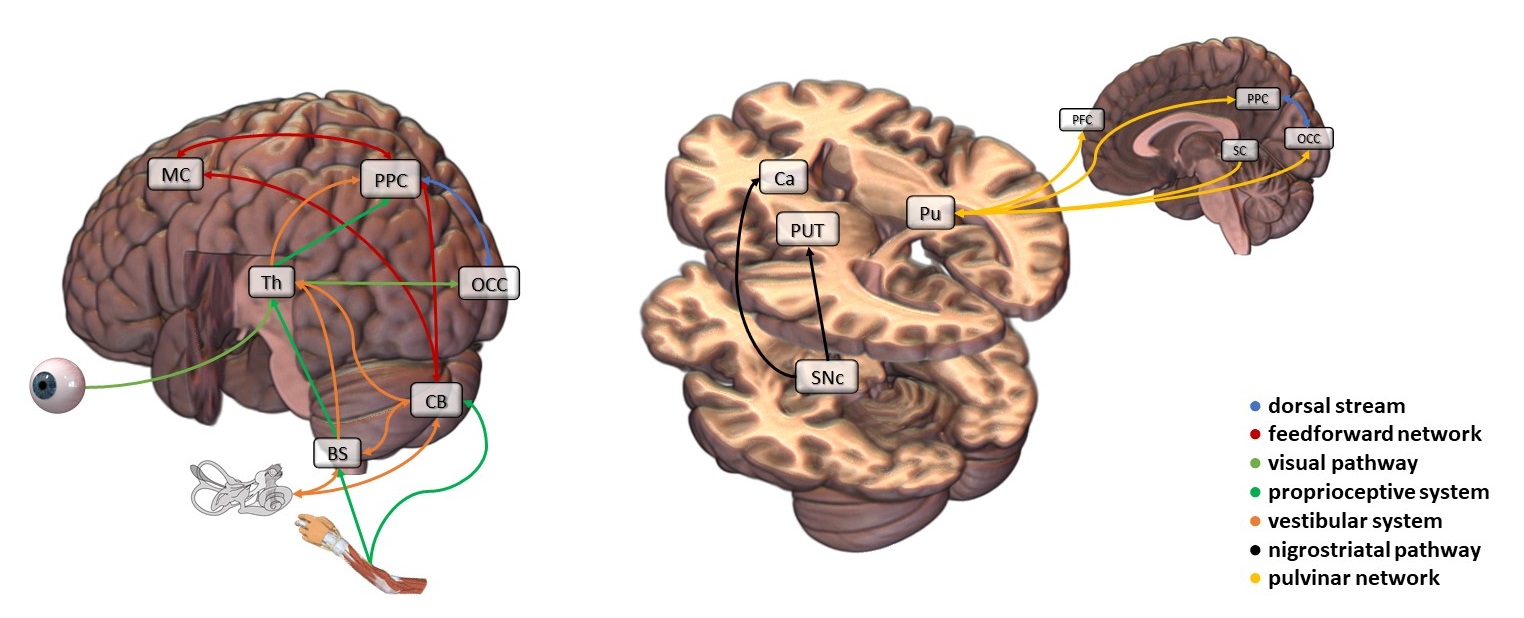
Neurophysiology of the main human brain networks involved in hand movements. Visual information travels from the retina to the primary visual cortex (V1). The axons of the retinal parvocellular/P-type ganglion cells project to the parvocellular layers of the dorsal lateral geniculate nucleus (LGN), those of the retinal magnocellular/M-type ganglion cells to the magnocellular layers. The pathway originating from neurons of the parvocellular LGN layers is specialized in the perception of colors, shapes, and details. Conversely, the pathway originating from neurons of the magnocellular LGN layers carries signals needed for analysis of speed and direction of moving stimuli, spatial location of objects in space, stereoscopic vision, and depth perception. Furthermore, it plays a pivotal role in guiding eye-hand movements. Indeed, at the cortical level, the magnocellular pathway is intimately interconnected with the occipito-parietal/dorsal stream, which is involved in planning and online control of hand movements. The dorsal stream uses visual information about the size, shape, and position of the target object to program and control movements in real-time according to egocentric coordinates, e.g., with respect to the observer's hand—Most of the efferent fibers from the vestibular nerve project towards the vestibular nuclei of the brainstem (BS), located between pons and medulla oblongata. Then, vestibular nuclei transmit information to the ventrolateral thalamic nuclei sorting it to the posterior parietal cortex (PPC). However, some vestibular neurons directly reach the cerebellum (CB). Vestibular nuclei and CB mutually exchange signals to regulate the antigravity muscles' tone and thus control balance. Note that any linear body movement or rotation instantaneously determines a concomitant movement of the visual image on the retina. Changes in visual information are also sent to the centers responsible for balance control. Accordingly, individuals suffering from a bilateral lesion of the vestibular organ might maintain balance almost normally on condition that they perform slow movements and that their eyes are both open—Proprioceptive information from muscles, tendons, and joints is largely transmitted to CB, although part of the proprioceptive signals reaches directly the PPC conveyed by the BS along the medial lemniscus (i.e., the medulla-thalamic circuit). Specifically, proprioceptive information reaches the nucleus gracilis and cuneatus and hence ascends to the ventrobasal complex of the thalamus (Th). Proprioceptive signals, together with tactile information, are integrated into the PPC, generating a conscious sense of position and movement of the different body parts in space—The CB is responsible for the muscular coordination of the distal portions of the limbs, with particular reference to hands and digits. Moreover, it guarantees harmonious progression from one movement to the next and the timing of every single movement during the execution of coordinated fine motor sequences but also when performing ballistic movements. The CB is the structural core of the feedforward network involved in the predictive phase of the action. Before starting a movement, the CB receives a copy of the motor command from the motor cortex (MC) and inputs from the parietal cortex. Based on sensory feedbacks (i.e., vestibular and proprioceptive signals) from the distal body parts, which communicate to the CB anterior lobe how the movement progresses, the CB executes a kind of “quality control” and can send rapid corrective signals to the cortex and spinal cord—The Pulvinar (Pu) plays an important role in filtering out irrelevant stimuli during the execution of movements. It has connections with both PPC and occipital cortex (OCC) and actively contributes, in concert with the occipitoparietal/dorsal stream, in performing visually-guided motor behaviors. The Pu contains retinotopic representations of the visual field and receives massive inputs from the superior colliculus (SC). The latter is involved in visuospatial attention, multisensory integration, and initiation/coordination of eye-hand movements—The nigrostriatal pathway connects the substantia nigra pars compacta (SNc) with the dorsal striatum, including the caudate nucleus (Ca) and putamen (PUT). SNc supplies dopamine to the striatum, which enhances the activity in the MC and reinforces adaptive motor programs, i.e., programs generating rewarding outcomes. Degeneration of the SNc dopaminergic neurons is one of the main pathophysiological features of Parkinson’s disease, characterized by rigidity, resting tremor, and akinesia. Indeed, a reduced supply of dopamine to the Ca may produce lower cognitive control of motor activity, i.e., the individual may show difficulties in responding to an external stimulus with rapid and appropriate intentional motor responses (e.g., reaching an object or regulating movement amplitude). In addition, decreased dopamine supply to the PUT may result in the inability to perform fine and sequential movements. Regarding the contents of the present caption, refer to Chieffi [157], Guyton [158], Guyton and Hall [159], Benarroch [160], and Knierim [161].