Academic Editor: Emilia Salvadori
Background: Blood flow restriction exercise (BFR-E) could be a useful
training adjunct for patients with weakness after stroke to augment the effects
of exercise on muscle activity. We aimed to examine neurophysiological changes
(primary aim) and assess patient perceptions (secondary aim) following BFR-E.
Methods: Fourteen participants with stroke performed BFR-E (1 session)
and exercise without blood flow restrictsion (Exercise only) (1 session), on two
days,
Strength training and repetitive task-specific training are important for regaining voluntary motor control, independence and promoting functional recovery after stroke [1, 2]. As recovery following stroke is time-dependent [3], it is important to ensure that the maximal possible training benefit is achieved in the least possible time, so that patients have time and opportunity to address any other impairments they may encounter following a stroke. Despite rehabilitation efforts, most stroke survivors do not fully regain strength and therefore face life-long disability [4]. Given this, strategies to enhance the efficiency and efficacy of strength training are essential to improve functional outcomes of people with stroke.
Blood flow restriction training is a training strategy used in healthy people, and has been proposed in elderly people, people following surgery [5] and neurological populations. The cardiovascular changes when exercising with blood flow restriction are widespread and include local and systemic mechanisms (see [6] for review). However, there is a complex interplay between the vascular and neurological system. Changing cardiovascular parameters can directly affect the metabolites within the blood and blood flow to the brain, via the blood brain barrier [7]. Furthermore, by occluding blood flow at the limbs, sensory and motor nerves are affected distal to the blood pressure cuff, and preferentially derecruited, depending on the cuff pressure and the length of time under blood flow restriction [8]. Given this, blood flow restriction, even though apparently influencing the cardiovascular system, can have widespread neurological effects.
In healthy elderly people, exercise regimes using blood flow restriction (BFR-E) increase muscle size, strength and function compared to Exercise only (E-only) regimes [9, 10, 11, 12]. Due to the proposed benefits, BFR-E has been prescribed for people following Anterior Cruciate Ligament (ACL)/orthopaedic surgery [13, 14, 15, 16], adults with osteoarthritis [15, 17], rheumatoid arthritis [15] and patellofemoral pain [14, 15, 16] to reduce pain and increase strength and function.
Although used in musculoskeletal populations, experimental studies applying BFR-E in people with stroke are sparse [18]. Despite this, a Japanese national survey showed that 11% of the Japanese rehabilitation clinics that use blood flow restriction (BFR), are using it in patients with stroke, presumably with the aim of optimising strength training and motor recovery [19, 20]. Moreover, BFR-E has also been investigated in people with other neurological disorders including Multiple Sclerosis [21, 22, 23, 24, 25] and incomplete Spinal Cord Injuries (SCI) [26, 27, 28, 29, 30, 31], with most (not all [24, 30]) studies showing that BFR-E was safe and increased muscle strength, size and function. One case study reported one event of symptomatic autonomic dysreflexia and three events of asymptomatic autonomic dysreflexia with BFR-E training in an individual with SCI, over a 4-week training period [30]. This case study highlights the importance of patient selection and screening, prior to commencing BFR-E, to ensure no pre-existing conditions are exacerbated by BFR-E [30]. Despite that study [30], in all other surveys/studies, BFR-E appears to be safe and well tolerated with very few adverse events reported.
In people with stroke, a small randomised controlled study, reported at a
conference, compared the effects of arm crank ergometry with and without BFR in
10 participants for 10 weeks 1
As neurological patients have been rarely investigated in BFR-E protocols, there is a lack of understanding on the neurological mechanisms underlying its potential effects in these patients. In healthy subjects, single sessions of BFR-E reduced single pulse motor evoked potentials (MEP)s in multiple muscle groups, for 20 to 30 minutes [32, 33, 34, 35, 36, 37, 38], indicating central fatigue. However, some studies have shown no post-exercise differences between BFR-E and E-only [39]. Paired pulse paradigms investigating short interval intracortical inhibition (SICI, interstimulus intervals of 2–3 ms) and intracortical facilitation (ICF, interstimulus intervals of 10–15 ms) have also shown no differences between BFR-E and E-only paradigms for SICI [39, 40] and ICF [39]. SICI can provide an indication of cortico-cortical inhibition due to Gamma-aminobutyric acid (GABA) mediated pathways [41, 42, 43, 44, 45] and while the mechanisms of ICF aren’t completely known it has been proposed that it provides an indication of the efficacy of cortico-cortical facilitation due to glutaminergic pathways [46, 47, 48, 49].
Although previous transcranial magnetic stimulation (TMS) studies in healthy people showed minimal differences in SICI and ICF between BFR-E and E-only, there is little information about the cortical effects of BFR-E following a stroke. This is an area worthy of further investigation given that there are well-document changes to the balance of cortical facilitation and inhibition after stroke [49]. For example, people with stroke have shown impaired GABA regulation and reduced GABA mediated inhibition. Furthermore, in the early phases of rehabilitation there is less SICI in the ipsilesional [49, 50] and contralesional hemispheres [46], where more GABA mediated disinhibition in ipsilesional and contralesional hemispheres appear to favour functional recovery [50, 51]. As high pressure BFR alone can temporarily reduce cortical GABA concentrations [52] and increase the size of motor volleys to deafferented muscles [53], it is possible that BFR could be used as a primer to alter cortical excitability and may augment the benefits of training/exercise. Moreover, chronic stroke patients, whose GABA concentrations reduced during E-only, showed greater improvements in function following 2 weeks of constraint induced movement therapy [54]. As such, it is possible that exercise in combination with BFR could assist in reducing GABA mediated inhibition and facilitate motor recovery. Although current evidence suggests that ICF does not change following stroke [49, 51], it can change following exercise (independent of BFR) in people with chronic stroke [55] and healthy people [39].
Considering the potential benefits of BFR-E in optimising muscle strength and
recovery after stroke, the purpose of the current study was to trial a low
pressure (0.8
The primary aims were to assess if the muscle activity was different between BFR-E and E-only during exercise and to determine if post-exercise neurophysiological measures (single pulse TMS, SICI, ICF and M-max amplitude) were different between 1 session BFR-E and 1 session E-only. The secondary aim was to determine if patient perceptions of pain, discomfort, fatigue, safety, focus and difficulty of exercise were different between BFR-E and E-only to ultimately assess if BRF-E is feasible and tolerated in stroke populations. To our knowledge, this is the first study to specifically train the ankle dorsiflexors in people with stroke using BFR-E. For the adoption of BRF-E into rehabilitation practice, we must understand whether participants will tolerate and be accepting of this training modality.
We consecutively recruited stroke patients over a 13 month period from October 2016 to November 2017. For this preliminary study, our recruitment target was 16 patients [56]. This sample size would provide us with an indication of neurophysiological effects of BFR-E as well as provide information about the feasibility and tolerability of BFR-E. Fifteen participants were recruited and 14 participants completed training (see Table 1 for participant characteristics).
Patient ID | Age | Sex | Time since lesion (days) | Type of stroke | Lesion location | Aff leg | Sys-BP (mmHg) | Dia-BP (mmHg) | Height (cm) | Mass (kg) | 10MWT (s) | MAS PFs/DFs | MMT PFn/DFn | TMS/Estim |
1 | 57 | F | 28 | I | MCA | R | 142 | 90 | 169 | 67 | 11.4 | 1+/0 | 5/4 | –/+ |
2 | 65 | F | 540 | I | MCA | R | 124 | 68 | 168 | 83 | 9.14 | 3/0 | 5/4 | –/+ |
3 | 58 | F | 25 | I | Pons | L | 120 | 74 | 160 | 76 | 7.85 | 2/0 | 5/5 | –/+ |
4 | 79 | M | 293 | H | MCA, Th | R | 133 | 63 | 178 | 80 | 19.95 | 2/0 | 4+/4+ | +/+ |
5 | 65 | M | 206 | I | MCA | R | 150 | 90 | 171 | 83 | 12.73 | 1/0 | 4+/4 | +/+ |
6 | 62 | F | 123 | H | F-lobe | R | 118 | 88 | 178 | 87 | 9.88 | 0/1 | 4+/4+ | +/+ |
7 | 59 | F | 113 | I | BG | L | 128 | 63 | 172 | 65 | 12.21 | 1+/0 | 5/4+ | +/+ |
8 | 64 | M | 430 | I | IC | R | 140 | 88 | 173 | 85 | 11.64 | 1+/0 | 5/4+ | –/+ |
9 | 55 | F | 66 | I | MCA | R | 124 | 74 | 164 | 93 | 23.75 | 1+/0 | 4/3+ | –/– |
10 | 36 | F | 400 | I | BG | R | 118 | 90 | 169 | 87 | 9.87 | 3/0 | 4+/4 | +/+ |
11 | 57 | M | 418 | I | BG | R | 124 | 66 | 180 | 90 | 10.44 | 1+/0 | 4+/5 | –/+ |
12 | 52 | M | 196 | I | BG, Pons | R | 126 | 84 | 183 | 95 | 9.21 | 2/0 | 5/4+ | +/+ |
13 | 47 | M | 118 | I | MCA | L | 124 | 74 | 183 | 99 | 6.63 | 1+/0 | 5/5 | +/+ |
14 | 62 | M | 431 | I | Th | R | 120 | 80 | 172 | 76 | 13.38 | 1+/0 | 5/4+ | +/+ |
F, Female; M, Male; I, ischemic; H, haemorrhagic; R, Right; L, Left; MCA, Middle Cerebral Artery; Th, Thalamus; F-lobe, Frontal lobe; BG, Basal Ganglia; IC, Internal Capsule; Sys-BP, Systolic blood pressure; Dia-BP, Disatolic blood pressure; 10MWT, 10 meter walk test time; MAS, Modified Ashworth Scale; MMT, Manual muscle Test; PFs, Plantarflexors; DFs, Dorsiflexors; PFn, Platarflexion; DFn, Dorsiflexion; TMS, Transcranial Magnetic Stimulation; Estim, Peripheral Electrical Stimulation; ‘+’, performed; ‘–’, not performed. |
Participants were included if they (a) were 18 to 80 years old, (b) had been
discharged from acute hospitalisation, (c) had a first time ischemic or
haemorrhagic stroke of any chronicity, (d) could walk 10 m with or without a
walking aid, (e) had manual muscle test scores of
Surface electromyography activity (sEMG) were recorded using (Ambu Neuroline)
surface electrodes. Electrodes were placed on the muscle belly of the tibialis
anterior in accordance with previous recommendations [57]. sEMG data were
amplified using custom built amplifiers and band pass filtered from 10 Hz to 500
Hz recorded at a sampling rate of 4 kHz. TMS was delivered using a magnetic
stimulator (Magstim 200, Magstim Company Ltd, United Kingdom) using a 110 mm
double cone coil. Data were collected and stimuli were controlled using
custom-made software (Mr Kick II) as has been used previously [58]. Brachial
blood pressure was measured using a sphygmomanometer (Riester® 55
cm
TMS and peripheral electrical stimulation were collected prior to exercise (T0), immediately after exercise (T1), 10 min after exercise (T2) and 20 minutes after exercise (T3). During testing at each time, peripheral electrical stimulation measurements always preceded TMS measurements.
The process for establishing thresholds and determining peripheral electrical
stimulus intensity has been described elsewhere [39, 58]. Briefly, 100
The process for establishing thresholds and determining TMS stimulus intensity
has been described elsewhere [39, 58]. Briefly, patients were lying and wore a
non-stretchable fabric cap that remained on the head during testing. Current was
applied in the anterior to posterior direction. The approximate hotspot was
determined by systematically moving and stimulating locations on the scalp (every
5–7 seconds) at the approximate location of the hotspot. To find the hotspot, an
intensity of 50% of the maximal stimulator output (MSO) was initially used. As some
patients had no discernible MEPs at 50% of the MSO in any scalp location, the
process was repeated for stimulus intensities of 55% and 60% of the MSO. If a
patient had no discernible MEP at 60% of the MSO, no further TMS was performed,
but patients continued with peripheral electrical stimulation and exercise. This
is because the paired pulse stimulation protocol for patients with resting motor
thresholds rMT(s)
Patients provided a numerical rating for their perceptions of pain, discomfort, difficulty and fatigue during exercise with 0 indicating no pain, discomfort or fatigue and 10 indicating the highest imaginable pain, discomfort or fatigue. Patients were also asked about how safe and focused they felt during exercise with 0 indicating feelings of being unsafe and unfocussed and 10 indicating feelings of being safe/focused.
Fig. 1 provides an overview of the experimental procedures. Prior to day 1, an
investigator explained the experimental procedures to participants via phone or
in person and participants were sent/provided a copy of the participation
information sheet and consent form. On day 1, an investigator discussed the
project with the participant and acquired written informed consent. In the
initial consultation and prior to testing, participants were screened against the
inclusion and exclusion criteria. Demographic and clinical data were collected
and included: age, sex, time since stroke, stroke location, dominant leg, height,
mass, 10-meter walk test time, modified Ashworth score of the plantarflexors and
dorsiflexors (in lying with the knee straight), manual muscle testing of the
plantarflexors and dorsiflexors (in lying with the knee straight) and brachial
blood pressure (measured on both days in lying on the less affected arm). If
participants were not suitable for testing based on these measures, no further
questioning/testing was performed. Following testing and after eligibility was
determined, the session performed first (BFR-E or E-only) was drawn randomly from
an envelope. Depending on randomization, participants performed E-only or BFR-E
in the first session, with the other condition performed in the second session.
Sessions were spaced 7
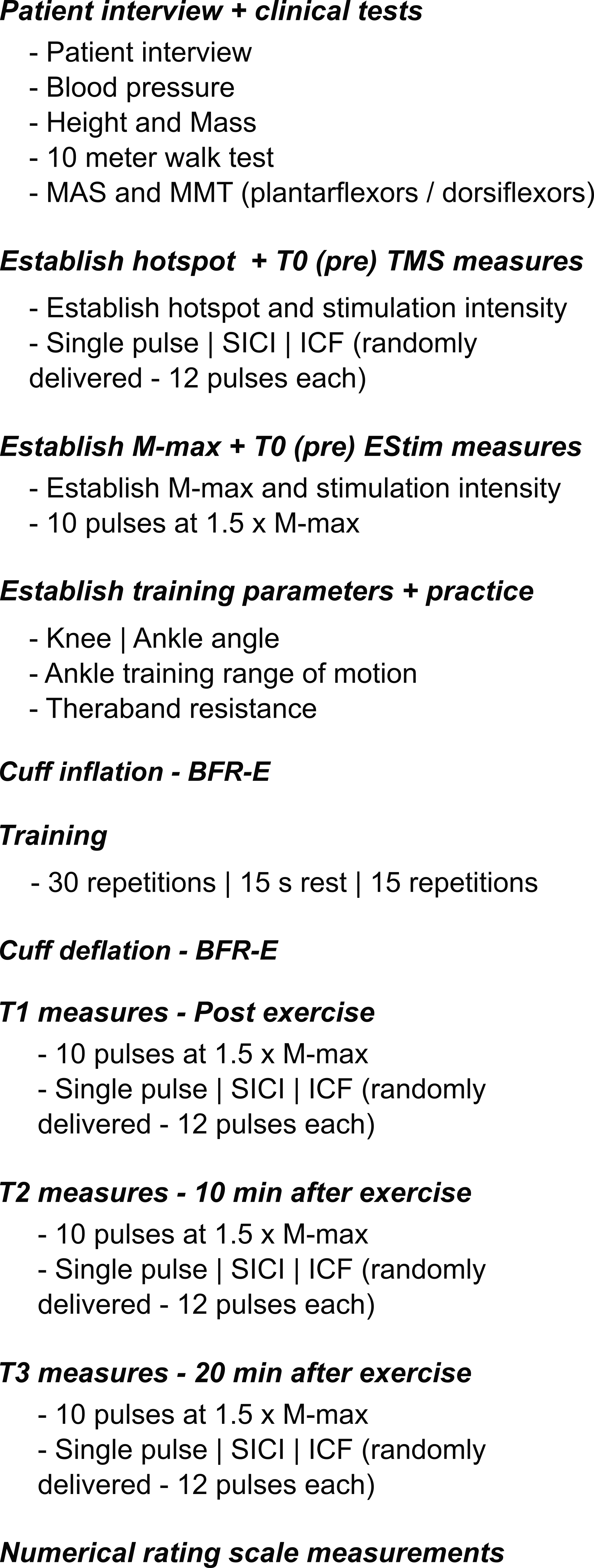
Overview of the experimental procedures. Abbreviations included in the figure: MAS, modified Ashworth scale; MMT, Manual Muscle test; M-max, Maximal peak-to-peak M-wave; EStim, Peripheral electrical stimulation; SICI, Short interval intracortical inhibition; ICF, Intracortical Facilitation; BFR-E, Exercise with Blood Flow Restriction; E-only, Exercise without Blood Flow Restriction; rMT, resting Motor Threshold.
After thresholds had been established, T0 measurements for TMS and peripheral electrical stimulation were collected. For all participants, sEMG electrodes were affixed and sEMG data were collected during training.
During training, patients were seated comfortably. The foot was relaxed and
resting on a board at an average ankle angle of 118
During training, participants performed one set of 30 repetitions and one set of
15 repetitions of dorsiflexion, with 30 seconds rest between sets. This is a
reduced exercise paradigm from blood flow restriction paradigms that have been
used previously [39, 40, 59, 60]. Participants concentrically contracted the TA
for
For BFR-E, the blood pressure cuff was placed around the thigh and inflated over
1 min to 0.8
Following training, participants that were eligible for TMS and M-wave measurements received these at T1, T2 and T3. Participants that did not received these, waited for 20 min before answering the numerical rating scales of the exercise session. For these questions, patients were asked only to comment on the exercise itself and not the neurophysiological testing, if performed.
For sEMG during training, the amplitude of the sEMG measurements during exercise
for each contraction were measured. Post data collection, data were smoothed
using a 1st order, 1 Hz low pass Butterworth filter. Contraction onset threshold
was 25
The amplitude of M-wave and MEPs was taken as the peak-to-peak amplitude
(
Data were assessed for normality using the Shapiro-Wilk test. Statistical tests appropriate to distribution were performed.
For sEMG data during exercise, a linear mixed model with subject and subject
For M-max and MEP data, for each time, each condition and each subject the (a)
average M-wave peak-to-peak amplitude was calculated, (b) average single pulse
TMS peak-to-peak amplitude was expressed as a percentage of the average M-max
peak-to-peak amplitude, (c) average SICI peak-to-peak amplitude was expressed as
a percentage of the average single pulse TMS peak-to-peak amplitude and, (d)
average ICF peak-to-peak amplitude was expressed as a percentage of the average
single pulse peak-to-peak amplitude. Linear mixed models with subject and subject
For the numerical rating scale data, Wilcoxon signed ranks tests compared BFR-E and E-only data for pain, discomfort, difficulty, safety, focus and fatigue.
When appropriate, means and 95% confidence intervals or median and
interquartile ranges were reported. Significance was set to p
Fifteen participants were recruited for the study. One participant was excluded
from the study after completing day 1. For this participant, on day 2, prior to
any testing, the systolic brachial blood pressure was
The remaining 14 participants are described in Table 1. All participants had post-exercise numerical rating scores and during exercise sEMG data for both BFR-E and E-only days. We had complete TMS and M-wave data for eight participants, complete M-wave data but no TMS data for a further five participants and no TMS and M-wave data for one participant. Four participants had no discernible MEPs at 60% of the MSO on day 1 when establishing TMS thresholds and were not tested further using TMS. One participant had discernible MEPs at 60% of the MSO on day 1 but not on day 2, and all TMS data for this participant were removed. One participant felt uncomfortable with electrical stimulation and TMS, and declined to have TMS and peripheral electrical stimulation.
Fig. 2 shows example rectified and smoothed data for one participant during exercise in BFR-E and E-only conditions.
During exercise there was a significant main effect of contraction-block
(p
Contraction block (estimate (95% CI)) | ||||||||||
Condition | C1 | C2 | C3 | C4 | C5 | C6 | C7 | C8 | C9 | |
Contraction amplitude, RMS | BFR-E | 116 (91 to 141) | 107 (82 to 132) | 104 (79 to 129) | 105 (80 to 130) | 103 (78 to 128) | 103 (78 to 128) | 113 (88 to 138) | 106 (81 to 131) | 107 (82 to 132) |
E-only | 111 (86 to 136) | 101 (76 to 126) | 98 (73 to 123) | 94 (69 to 119) | 94 (69 to 119) | 93 (68 to 118) | 106 (81 to 131) | 93 (68 to 118) | 91 (66 to 116) |
Main effect (contraction block) (minus C1) | Main effect (condition) | ||||||||
C2 | C3 | C4 | C5 | C6 | C7 | C8 | C9 | BFR-E minus E-only | |
mean difference (95% CI) | mean difference (95% CI) | mean difference (95% CI) | mean difference (95% CI) | mean difference (95% CI) | mean difference (95% CI) | mean difference (95% CI) | mean difference (95% CI) | mean difference (95% CI) | |
Contraction amplitude, RMS | –9 (–13 to –5)* | –12 (–16 to –8)* | –14 (–18 to –10)* | –15 (–19 to –11)* | –15 (–19 to –11)* | –4 (–8 to 0)* | –14 (–18 to –10)* | –15 (–19 to –11)* | 9 (–11 to 29) |
* represents significant differences to p |
Fig. 3 shows example raw data for one participant for M-max, single pulse TMS, SICI and ICF stimulation paradigms before and after BFR-E and E-only conditions.
There was no significant condition
Variable | Condition | T0 — estimate (95% CI) | T1 — estimate (95% CI) | T2 — estimate (95% CI) | T3 — estimate (95% CI) |
M-max amplitude, µV | BFR-E | 2307 (1877 to 2737) | 2129 (1699 to 2559) | 2184 (1754 to 2614) | 2234 (1804 to 2664) |
E-only | 2384 (1954 to 2814) | 2366 (1936 to 2796) | 2307 (1877 to 2727) | 2419 (1989 to 2849) | |
Single pulse amplitude, % of M-max | BFR-E | 20 (13 to 27) | 20 (13 to 27) | 21 (14 to 28) | 20 (13 to 27) |
E-only | 19 (12 to 26) | 20 (13 to 27) | 22 (15 to 29) | 25 (18 to 32) | |
SICI amplitude, % of single pulse amplitude | BFR-E | 74 (59 to 89) | 74 (60 to 89) | 76 (61 to 91) | 71 (57 to 86) |
E-only | 74 (59 to 89) | 74 (59 to 89) | 80 (65 to 94) | 74 (59 to 89) | |
ICF amplitude, % of single pulse amplitude | BFR-E | 188 (81 to 295) | 187 (80 to 294) | 164 (57 to 270) | 161 (55 to 268) |
E-only | 305 (198 to 412) | 296 (189 to 403) | 224 (117 to 331) | 255 (149 to 362) | |
All values are rounded to whole numbers; T0 = pre-exercise; T1 = immediately post-exercise, T2 = 10 min post-exercise; T3 = 20 min post-exercise. |
Variable | Main effect (time) | Main effect (condition) | ||
T1 minus T0 | T2 minus T0 | T3 minus T0 | BFR-E minus E-only | |
mean difference (95% CI) | mean difference (95% CI) | mean difference (95% CI) | mean difference (95% CI) | |
M-max amplitude, µV | –98 (–205 to 9) | –100 (–207 to 7) | –19 (–126 to 87) | –156 (–470 to 159) |
Single pulse amplitude, % of M-max | 1 (–2 to 4) | 2 (–1 to 6) | 4 (0 to 7) | –1 (–5 to 3) |
SICI amplitude, % of single pulse amplitude | 0 (–10 to 11) | 4 (–6 to 14) | –1 (–11 to 9) | –2 (–10 to 7) |
ICF amplitude, % of single pulse amplitude | –5 (–74 to 64) | –53 (–121 to 16) | –38 (–107 to 31) | –95* (–165 to –25) |
All values are rounded to whole numbers; * represents significant differences to
p |
Table 6 summarises the numerical rating scores for BFR-E and E-only. There were no differences between conditions for patient perceived pain (p = 0.10), discomfort (p = 0.17), fatigue (p = 0.47), safety (p = 0.50), focus (p = 0.24) or difficulty (p = 0.16).
BFR-E | E-only | |
median (IQR) | median (IQR) | |
Pain | 0 (0–1) | 0 (0–0) |
Discomfort | 0 (0–1) | 0 (0–1) |
Fatigue | 3 (1–4) | 2 (1–4) |
Safety | 10 (10–10) | 10 (9–10) |
Focus | 10 (8–10) | 10 (9–10) |
Difficulty | 1 (0–6) | 1 (0–3) |
Numerical rating scores rounded to whole numbers. |
One session of dorsiflexion resistance
exercise has not previously been compared between BFR-E and E-only conditions in
participants following stroke. We found no contraction-block
There were no interaction effects for single pulse stimulation, SICI and ICF. Although a main effect of condition was reported for ICF, this is a reflection of the higher ICF at baseline. Unfortunately, we could only find rMT in eight participants. For five participants the rMT could not be found on both days. Following stroke, it is common for patients to have reduced cortical excitability in the affected hemisphere compared to the unaffected hemisphere and compared to healthy controls [49]. A systematic review demonstrated large standardised main differences (SMD) and relatively narrow 95% confidence intervals (95% CI) when comparing the affected hemisphere with the unaffected hemisphere for rMT (SMD: 1.03, 95% CI: 0.84 to 1.23) and MEP amplitude (SMD: –0.96, 95% CI: –1.17 to –0.74) and the affected hemisphere with healthy controls for rMT (SMD: 1.24, 95% CI: 0.81 to 1.67) and MEP amplitude (SMD: –0.64, 95% CI: –0.93 to 0.34) [49]. These differences were apparent in both early and chronic phases of stroke [49]. Given this, the inability to determine a rMT in five participants, may not be unexpected.
When determining our target sample size of 16, we expected that although rMT may
be higher and MEP amplitude maybe reduced in our cohort, we would still be able
to attain a rMT. It is difficult to ascertain why our cohort had so many
non-responders when patients were relatively well functioning and had a
dorsiflexor MMT strength of
A further consideration is that the responses from patients in our study may have been too variable to observe a consistent effect. The patients differed in the time since stroke, location of stroke and type of stroke, which can increase inter-subject variability of neurophysiological responses [46]. In our analysis, we adjusted for time since lesion (as a continuous variable) and found no effect for any neurophysiological measure. We did not add location of stroke or stroke severity as a factor as we did not have sufficient patient numbers with each to do this however a cross over design was chosen to reduce some of this variance.
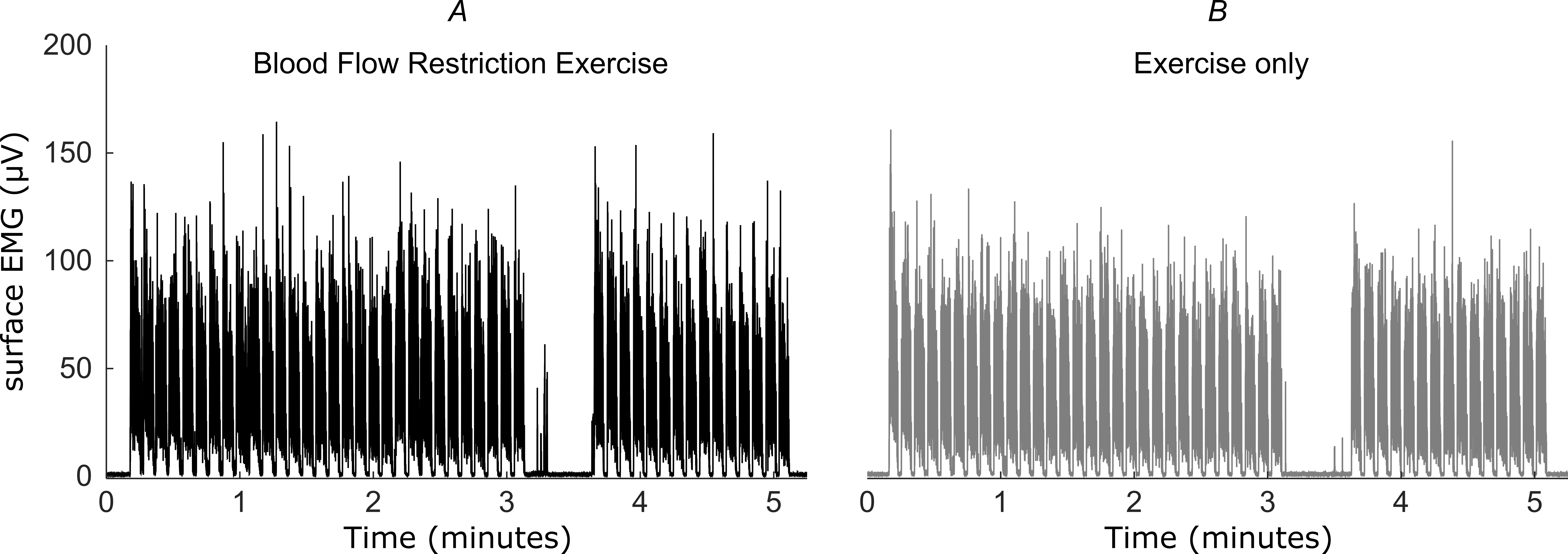
Example traces of the rectified and smoothed (20 Hz low
pass Butterworth filter) surface EMG (
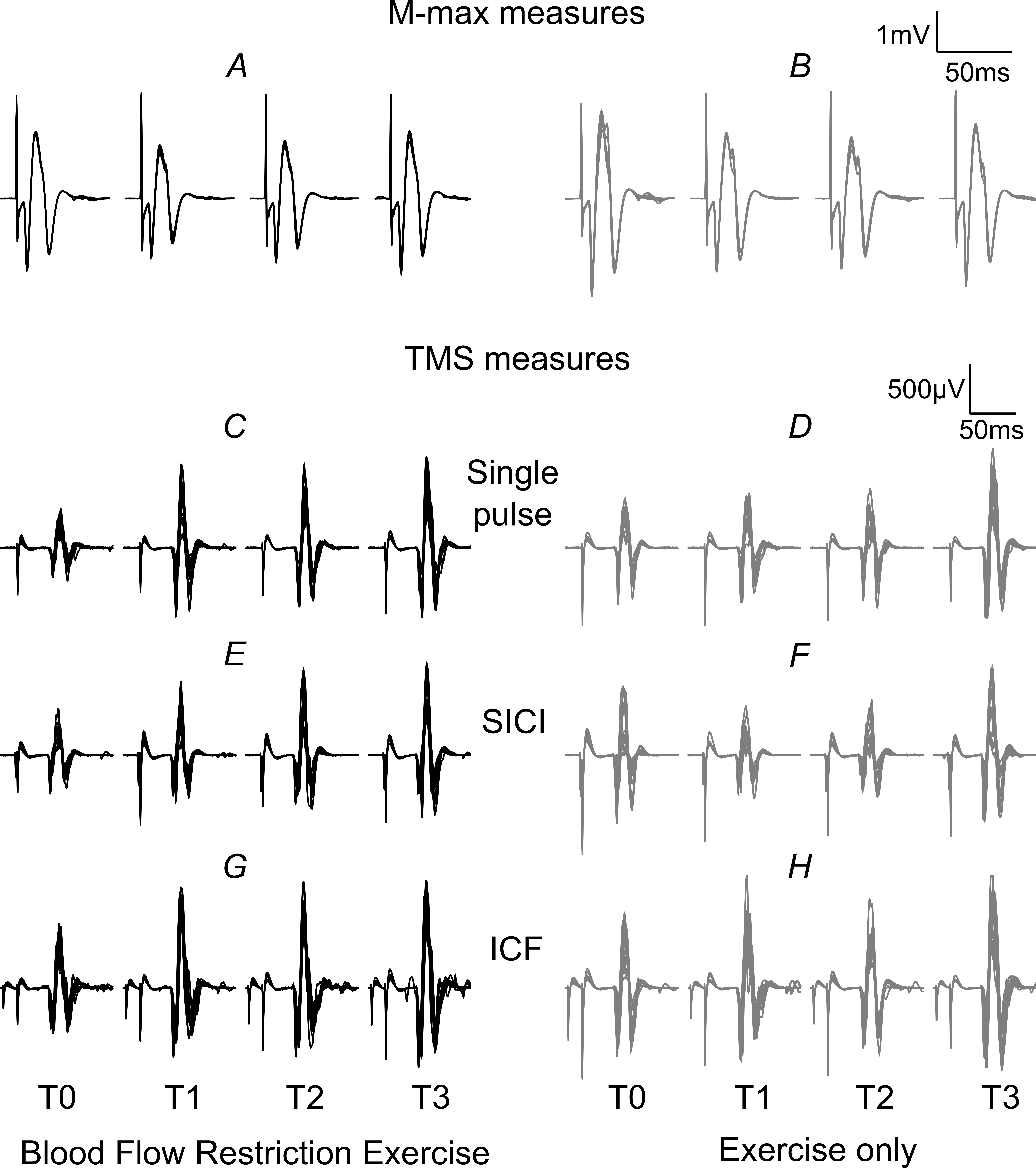
Example traces of raw surface EMG (
Taken together, although we almost attained our target of 16 participants, due to the number of non-responders and response variability, our study was likely underpowered to determine changes/differences in TMS measurements if a difference exists. While we acknowledge that this is a significant limitation, non-publication of non-significant results, and ‘file-drawer’ publications, has been highlighted as a significant problem in TMS research [65]. As such, we decided to disseminate our results rather than not disseminate the results at all.
Although potentially underpowered, it is also possible that there is little
difference in TMS measurements with exercise (without BFR). Although E-only
paradigms in healthy subjects have shown SICI disinhibition [66, 67, 68], some have
not [69]. Further, in people with chronic stroke SICI in the abductor pollicis
brevis was not different for all time points after training following a single 15
min bout of repetitive thumb abduction training [55]. Our exercise protocol was
shorter compared to these paradigms and perhaps too short to induce changes in
SICI. Further, our paradigm was in lower limb muscles which can alter projections
to muscles [70]. Similarly to SICI, there was no significant interaction in ICF
between time and BFR-E and E-only conditions. Although previous studies have shown changes
in ICF following exercise in healthy people [39, 68, 69] and stroke patients [55],
not all have in E-only [39] or BFR-E [39] conditions. The result of our study
could mean that SICI and ICF in people with stroke are not different when
comparing BFR-E and E-only. Alternatively, the lack of difference between the
BFR-E and E-only could be that the exercise intensity was too low to result in
sufficient fatigue in the BFR-E condition and/or occlusion pressures used were
too low to influence SICI. Studies that have demonstrated altered SICI as a
result of BFR-E, have used significantly higher pressures for longer periods of
time (
Safety and comfort are important aspects when assessing feasibility of new rehabilitation strategies before designing larger studies to test its effectiveness [72]. In our study, patients felt safe during both interventions with no difference between interventions. In addition, subjects reported minimal discomfort with no reported or observed adverse reactions. Although this is encouraging, based on our findings we do not believe that BFR-E should be used in all people with stroke, in all settings. Participants were selected based on a stringent inclusion and exclusion criteria and may not represent all people following stroke. In this study, exercise was overseen by a physiotherapist in an inpatient hospital with a neurologist on standby and a local medical emergency response team in case of emergency, which does not reflect all clinical settings. In our sample, participants perceived exercise as minimally fatiguing. However, in stroke populations with different inclusion/exclusion criteria, with more fatiguing exercises over more sessions, it is possible that adverse reactions are more likely to occur. Previous research has shown that the frequency of self-reported fatigue affects 69.5% of people 1-year following stroke [73] and is 68% more prominent in people with stroke than healthy people [74]. If similar studies are performed in the future, we recommend that this is considered when choosing exercise intensity and training setting.
Patients reported minimal pain and discomfort and no difference in pain and discomfort between BFR-E and E-only conditions. This is encouraging as people with stroke are more likely to comply with exercise that doesn’t cause pain or discomfort [75]. However, it also highlights that exercise was (perhaps) too easy or that cuff pressures were too low for most patients as some pain/discomfort is expected during BFR-E [58]. Future studies should consider increasing exercise intensity and cuff pressures during BFR-E to potentially increase the effects of BFR-E.
In this study, participants generally had few comorbidities and high mobility (i.e., could walk 10 meters with or without walking aids), which is not representative of many people following stroke who are weaker and/or lower functioning. Although the participants included in our study were relatively well-functioning, as we included a convenience-based sample, there was some heterogeneity in the included population. For example, some patients received thrombolysis on admission to acute care (subject 10 and 11 received thrombolysis, subject 8 was missing and the remaining did not receive thrombolysis) and some had depression (subject 3, 8 and 14). Unfortunately, we were not able to attain the medication status, did not measure/attain general post-stroke fatigue nor have reliable/valid data on thrombectomy. These are factors that can influence neural recovery and peripheral responses to TMS. Furthermore, although all patients included in the study had deficits as a result of the stroke (as this was one of our questions in the initial interview), we cannot definitively rule out that some of the participants may have had existing issues which further exacerbated the impairment caused by the stroke. It would have been ideal to have medication status, post stroke fatigue, thrombectomy data and pre-existing deficits. However, as we used a cross-over type design and accounted for this in our analysis, although a limitation, it is part of the difficulties when conducting research in an inpatient setting and using a convenience based sample. Exercise was performed in an inpatient hospital with significant safety measures in place, should a patient experience distress. Future research using similar paradigms must account for this to ensure safety of patients when performing BFR-E. Furthermore, our training protocol was short, over 1 session, and does not account for the amount of practice required for functional recovery after stroke nor the reality of most clinical settings.
To our knowledge, this is the first study to specifically train the ankle
dorsiflexors in stroke patients using BFR-E. Although 14 participants completed
the study protocol, only eight participants completed TMS measures on both
days. Post exercise SICI, ICF and single pulse TMS showed no interaction effect
for conditions
SICI, Short interval intracortical inhibition; ICF, Intracortical Facilitation; MEP, Motor Evoked Potential; BFR-E, Exercise with Blood Flow Restriction; E-only, Exercise without Blood Flow Restriction; EMG, Electromyographic activity; rMT, resting Motor Threshold; MSO, Maximal Stimulator Output.
Conceptualization—ETNS, JFN, PWS; Project Administration—SSK, PWS; Methodology—SSK, ETNS, JFN, PWS; Resources—ETNS, JFN; Investigation—ETNS, SSK, PWS; Formal Analysis—PWS; Interpretation of data—SSK, ETNS, ML, CQdO, JFN, PWS; Visualization—PWS; Writing—Original Draft Preparation—SSK, ETNS, ML, CQdO, JFN, PWS; Writing—Review & Editing—SSK, ETNS, ML, CQdO, JFN, PWS.
The study conformed to the Declaration of Helsinki and was approved by the local scientific ethics committee (approval number: 1-10-72-279-14) and the Danish Data Protection agency (approval number: 1-16-02-520-14). Participants were recruited via an inpatient rehabilitation ward or had provided details in previous studies allowing researchers to contact them for future studies. All subjects provided written informed consent prior to undertaking the study.
We would like to thank the participants for being part of this study.
This study was supported by a grant (project nr. 614864) from the Health Research Fund of the Central Denmark Region to Peter Stubbs.
The authors declare no conflict of interest.