†These authors contributed equally.
Academic Editor: Roman R. Poznanski
Thyrotropin-releasing hormone (TRH) and its receptors are expressed in the hypothalamus and limbic regions. Brain thyrotropin-releasing hormone actions are exerted directly through its receptors and indirectly by modulating the effects of neurotransmitters such as glutamate, gamma-aminobutyric acid, acetylcholine, and dopamine. The thyrotropin-releasing hormone has been implicated in eating and mood regulation. We integrate studies that analyze the role of limbic thyrotropin-releasing hormone on displaying depressive- and anxiety-like behaviors and anorexia or hyperphagia. Since the decade of 1970s, different efforts have been made to identify some of the thyrotropin-releasing hormone effects and its analogs in feeding regulation or to ameliorate symptoms in patients diagnosed with mood disorders, and to correlate anxious or depressive parameters with thyrotropin-releasing hormone levels in the cerebrospinal fluid or its expression in postmortem brain areas of affected patients. Pharmacological studies where the thyrotropin-releasing hormone is administered to animals by different routes and to distinct brain areas have elucidated its actions in behavioral changes of mood and feeding parameters. In addition, a variety of animal models of depression, anxiety, or anorexia and hyperphagia has suggested the association between the hypothalamic and limbic TRHergic system and the regulation of mood and feeding alterations. Different approaches employ the administration of anti-depressant, anxiolytic or anorectic agents to animals and describe changes in thyrotropin-releasing hormone content or expression in hypothalamic or limbic regions. The different effects on mood that result from modulating thyrotropin-releasing hormone expression may be beneficial to treat patients diagnosed with eating disorders.
The thyrotropin-releasing hormone (TRH) was the first hypothalamic peptide [1]
identified and described as a neurohormone responsible for directing the function
of the hypothalamus-pituitary-thyroid (HPT) axis. TRH is a tripeptide amide
(pGlu-His-ProNH
The transcription of the TRH gene is regulated by response elements
present on its promoter. Some transcription factors positively regulate
prepro-TRH synthesis such as the glucocorticoid response element (GRE), cAMP
response element-binding protein (CREB), 12-O-Tetradecanoylphorbol-13-acetate
(TPA) response element for Jun/Fos (AP1), signal transducer and activator of
transcription (STAT): or negatively as the TH response element (TRE) [9]. TRH
exerts its effects through postsynaptic TRH-R1 and TRH-R2 receptors (not present
in humans). TRH-R1 receptor is the subtype mainly expressed in the anterior
pituitary. Thus it is the one involved in the role of TRH as a neurohormone.
However, TRH-R1 is also localized in central regions such as the Hyp hippocampus
(Hipp), amygdala (Amy), and nucleus accumbens (NAc). TRH-R2 receptors are located
in the prefrontal cortex (PFC), the PVN, and the Amy, among other regions. They
have been implicated in mediating TRH’s sensory and cognitive processes [10, 11, 12, 13, 14].
Both are G- protein-coupled receptors by activating G
Although the hypophysiotropic role of TRH in regulating the HPT axis has been thoroughly studied, its modulatory effects in mood-related behaviors are not fully understood. Different studies were trying to define whether TRH actions are directly executed on postsynaptic cells or indirectly by modulating the effects of different neurotransmitters (NT) from interneurons or presynaptic cells, supporting the idea of a neuromodulatory effect.
Direct actions of TRH on postsynaptic cells are inferred from in vitro studies showing a consistent response, which may be stimulatory or inhibitory of
cellular activity, depending on the brain area studied. This effect is
independent of the actions of interneurons and from the interference caused by
adding different NT or by lowering Ca
Early studies of TRH electrophysiological effects also support modulating the cellular responses in different brain areas induced by NT such as glutamate, GABA, acetylcholine, norepinephrine, dopamine (DA), and serotonin, among others. Overall, this supports the participation of TRH in several behaviors mediated by these NT.
DA is a neurotransmitter modulated by TRH, its administration in the NAc of rats seems to facilitate DA release by acting presynaptically in the DA-containing neurons arising from the ventral tegmental area; moreover, TRH is also able to potentiate DA-induced activation of cAMP levels in the DA postsynaptic cells in the dorsal part of the same region [19]. Other studies show that antagonizing type 2 DA receptors cause a blockage of the suppressing effect of TRH in rats’ preference for ethanol, demonstrating the importance of a functional dopaminergic system in mediating behavioral properties of TRH [20, 21].
Regarding GABAergic neurotransmission, TRH is known to depolarize thalamic GABAergic cells and to potentiate the antiepileptic and sleep actions of barbiturates; in contrast, in the Hipp TRH can depress the GABA-induced inhibitory postsynaptic potentials [22], which implicates the peptide in improving CA1 pyramidal cells regulation of memory processes. In cortical cells, TRH can potentiate excitatory actions of acetylcholine [23] or induce its release in rats’ cortex and Hipp [24]. These findings suggest that TRH modulates acetylcholine participation in cognitive functions.
Although findings are controversial regarding stimulatory or inhibitory actions of TRH on cortical cells’ responses to glutamate, all agree on its modulatory effects on the aminoacid excitatory actions [25, 26]. In contrast, in hippocampal neurons, TRH seems to induce biphasic effects: early after its administration, it depresses N-methyl-d-aspartate (NMDA) stimulatory actions, whereas, after prolonged times, TRH potentiates glutamate excitatory effects [27]. These neuromodulatory actions support TRH’s role in mood regulation or memory and learning processes.
Given the vast evidence of TRH modulation on NT actions and behaviors, we systematically review 98 articles to obtain an integrated analysis of the literature available to date regarding the association between hypothalamic and limbic TRH systems with mood and eating regulation.
To provide an overview of the evidence regarding the role of TRH in behaviors such as anxiety, depression and feeding, a series of systematic searches were performed in APA PsycInfo and MEDLINE Complete databases. We did not restrict the publications dates, and reviews were not considered. Three different searches were performed; the search strings consisted of (1) TRH or thyrotropin-releasing hormone and anxiety, (2) TRH or thyrotropin-releasing hormone and depression, (3) TRH or thyrotropin-releasing hormone and feeding, and their synonyms and equivalent subjects. These terms were combined with logical functions and operators using the “OR and AND” to narrow the search. We included preclinical and clinical studies, including. 711 papers on TRH and depression, 157 of TRH and anxiety, and 246 of TRH and feeding were obtained. Titles and abstracts were screened for a depuration of potentially relevant studies, and papers focused only on the HPT axis were not considered. Articles were chosen based on the following classifications: (1) TRHergic system in animal models, (2) Exogenous TRH administration and changes in behavior, (3) Exogenous drug administration and changes in endogenous central TRHergic system, and (4) Clinical studies. Full-text articles were retrieved and analyzed for relevant results, and if papers did not meet the classification criteria, they were not considered (Fig. 1, Ref. [28]).
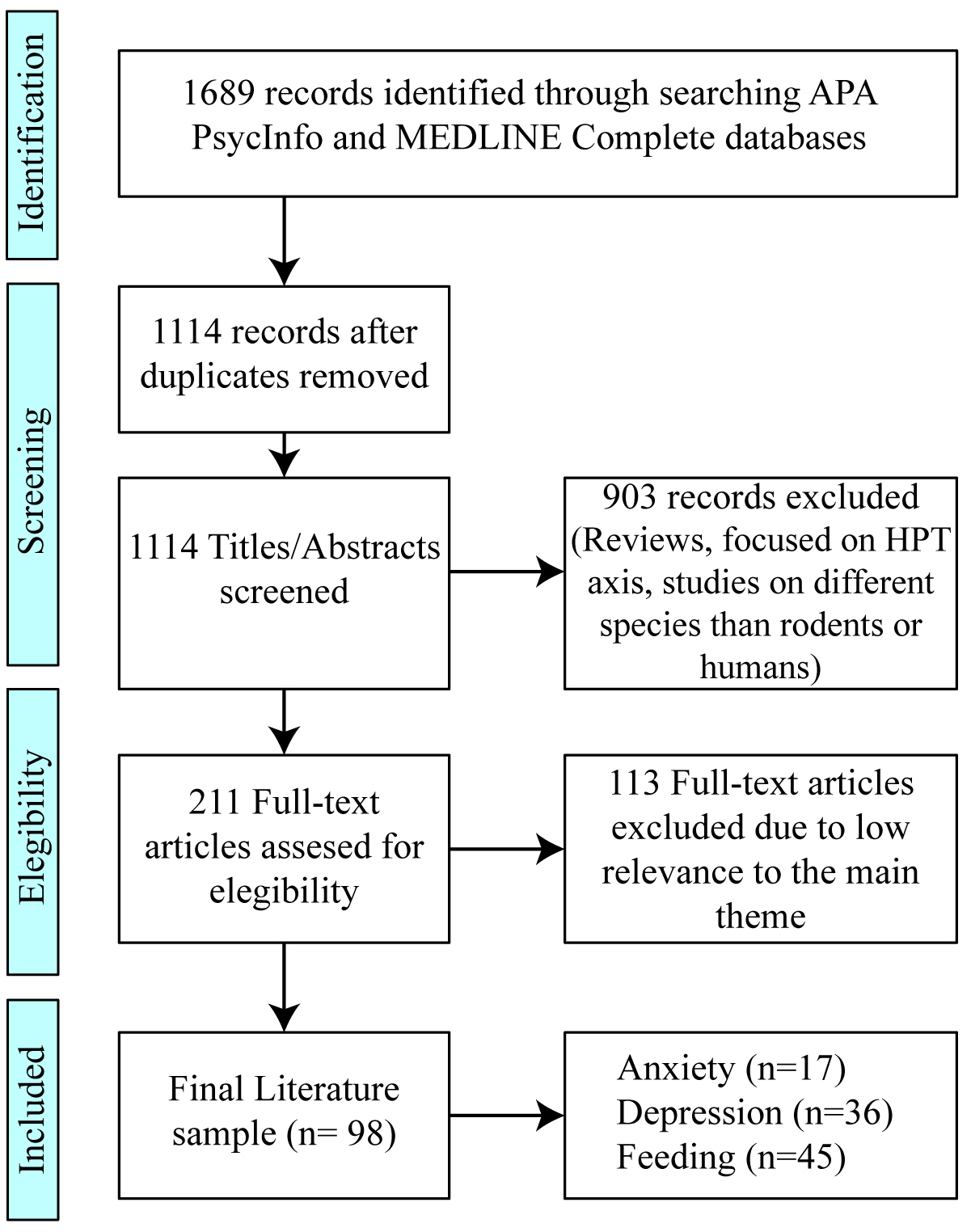
Flowchart of the search and selection process identification. In order to achieve high standard of reporting, the Preferred Reporting Items for Systematic Reviews and Meta-Analyses, (PRISMA) guidelines [28] were adopted.
The search yielded 157 results, and only 21 were considered potentially relevant for the analysis. Of these, 4 were excluded due to a failure to associate with anxiety or the analysis was based on TRH-like peptides only. Finally, 17 articles were used to analyze the data, and 16 were performed in rodents and one in humans (See Appendix Table 1).
Seven reports were included in this classification. Six studies contained data about TRH expression in the Hyp or limbic areas and behavioral tests such as the elevated plus-maze (EPM), the defensive burying test (DBT), the open field test (OFT), and the novelty-induced hypophagia test (NIHT), to analyze anxiety-like behavior in rodents (rats or mice). One report describes TRH content changes during the estrous cycle of female rats, given that estradiol is a neuroprotective hormone and its fluctuations have been associated with anxiety disorders. It shows that proestrus promotes a decrease in TRH content in Hyp, Amy, NAc and Hipp [29]. Three reports that use growth hormone-releasing hormone (GHRH) knockout (KO) mice [30], and Wistar or Wistar Kyoto (WKY) rats (strain with increased depression- and anxiety-like behaviors) [6, 31] find an inverse correlation between TRH mRNA expression either in the Hyp or in the Amy with anxiety-like behaviors. GHRH KO mice exhibit reduced anxiety in the OFT and the EPM and increased TRH mRNA expression in the Hyp [30]; whereas when rats are subjected to the DBT, increases in TRH content and decreases on its mRNA levels after 30 or 60 min of performing the test are observed in Amy of Wistar and WKY rats [6, 31]. These results support the anxiolytic role of TRH. The other three reports fail to find a clear association between changes in the TRH system and anxiety. In two different studies that use KO mice for TRH-R1 and TRH-R2 [32, 33], anxiety-like behavior is increased in TRH-R1 KO mice when performing the EPM and decreased in TRH-R2 KO female mice after subjecting them to NIHT. In a model of anorexia in rats [34], TRH mRNA expression increases in the PVN, but anxiety-like behavior increases in such female rats subjected to the DBT.
We included three studies where TRH was intracerebroventricularly (i.c.v.) or intraperitoneally (i.p.) administered, and the DBT and the EPM test anxiety-like behavior. One of those studies was also included in classification 1 [6]. According to these reports, there is controversy regarding TRH’s effects on anxiety. I.c.v. TRH administration to Wistar rats reduces anxiety in the DBT and decreases corticosterone serum levels [6].
On the other hand, in another report where TRH is i.c.v. Injected to Holtzman rats shows no effects on anxiety-like behavior analyzed with the EPM [35]. Likewise, an i.p. administration of TRH does not affect anxiety in rats subjected to the EPM [36]. More efforts are needed to discriminate TRH effects of different limbic regions on anxiety behavior.
Seven studies were included where the administration of different drugs or
hormones alters TRH expression or content in several brain regions. Two studies
evaluate TRH changes after administering anxiolytic drugs ketamine or prazosin,
glutamatergic or adrenergic neurotransmission inhibitors, respectively. The i.p.
injection of ketamine induces an increase in the content of TRH in the Hyp, NAc,
PFC and Amy of Sprague-Dawley rats [37], and the administration of prazosin
enhances TRH content in the Amy, NAc and striatum of Sprague-Dawley and Lewis
rats, a strain with high-extreme behavioral responses [38]. One report evaluates
the inhibition of TRH binding to its receptor by benzodiazepines (anxiolytic
drugs) and finds that in Amy, its receptor (not identified as its subtype at the
time) is more sensitive to the inhibitory actions of these drugs [39]. This
evidence suggests that TRH could be mediating the effects of anxiolytic drugs.
Two articles report changes in the early stages of life (gestation or neonatal)
on the TRH system and anxiety-like behavior displayed by rodents. Neonatal
serotonin depletion by administering para-chlorophenylalanine (pCPA) induces a
reduction in anxiety in the OFT, but TRH mRNA expression in the PVN does not
change [40]. On the other hand, oral administration of thyroxine (T
The administration of drugs that directly affect TRH transcription has also been evaluated. The role of TRH from the NAc in anxiety has been supported by the reduced anxiety-like behavior in the DBT, which is observed along with an upregulation of TRH expression after an intra-accumbal administration of an inhibitor of the enzyme phosphodiesterase 7 (PDE7B) in ad libitum fed rats. This approach maintains elevated phosphorylated CREB (pCREB) levels, enhancing TRH transcription. When an antisense oligonucleotide (ODN) is injected before administrating the PDE7B inhibitor, a decrease in accumbal TRH mRNA levels develops, and anxiety returns to the level of a vehicle-treated group. It is noteworthy that the i.p. injection of PDE7B inhibitor affects feeding and anxiety depending on the dose administered: while a lower concentration (0.2 mg) of PDE7 inhibitor increases the expression of TRH in the PVN and decreases feeding (without affecting anxiety-like behavior), a higher dose enhanced accumbal TRH mRNA and induces anxiolysis, with no changes in food intake [42]. Lastly, i.p., corticosterone administration induces TRH content in Hipp and Amy’s striatum [43]. Overall, we can conclude that TRH from limbic regions, especially from Amy and NAc, regulates mood and modulates anxiolytic drugs’ actions.
Only one report found that TRH content in the cerebrospinal fluid (CSF) was correlated to anxiety in diagnosed patients [44]. Although no statistical differences are found, a weak trend of a lower CSF TRH content in anxious patients is observed. This could be accounted for the small number of patients included in the report (45 patients). The lack of research of TRH association to anxiety in clinical studies shows that this field could be exploited further.
After analyzing 711 articles, we selected 36 studies. Research shows that the efforts to analyze TRH’s role in depression have been documented since the 1970s. In the early years, most research relied on evaluating TSH response after an intravenous (i.v.) injection of TRH (TRH stimulation test), where a blunted TSH release is consistently reported in depressed patients. However, we did not consider the articles focusing on an unresponsive thyroid axis’s association with an exogenous TRH administration. In contrast, we described the neuromodulatory role of the peptide in depression (See Appendix Table 1).
We found sixteen articles that analyze the participation of the peptide in the display of depression-like behaviors by using various models. Some of the most recent articles show that TRH may be a factor that facilitates depression-like behaviors in rats since TRH mRNA content and that of its receptor TRH-R1, which are both highly expressed in the cortico-limbic pathway, result upregulated in the basolateral Amy (BLA) of animals with chronic stress (2 h of restraint for 14 days). This TRH upregulation is observed along with high scores of depressive parameters when performing the forced-swim test (FST) or tail-suspension test (TST); in an elegant experiment, researchers show that siRNAs injection directed against TRH or TRH-R1 in BLA decrease depression-like conducts [45].
Also, TRH content and expression increase in the BLA of chronically stressed animals, which also present an up-regulation of interleukin-18 (pro-depressant factor) in this area and elevated immobility time when performing the FST and the TST. Interestingly, interleukin-18 KO mice and rodents with interleukin-18 silenced expression show low depressive parameters and fail to present the elevated TRH mRNA levels in BLA of wild-type stressed animals. Therefore, the amygdalar TRHergic pathway seems to be associated with high levels of depression and downstream of interleukin-18 signaling inducing depression-like behaviors in chronically stressed animals [46].
In WKY rats that develop a hypothyroideal condition and high immobility scores
in the FST, the prepro-TRH protein levels of Amy increase in gestational
T
Another approach is using acute or chronic corticosterone administration to rats, given its involvement in displaying depression in humans and animals. A single i.p. injection of a high dose of corticosterone elevates TRH content in the Hipp, Amy, entorhinal cortex and striatum of rats after 4 or 8 h of being injected [43]. In contrast, male rats ingesting the steroid in drinking water for 20 days, a condition that induces depression-like behaviors, show decreased TRH content in the Amy or PFC, depending on low or high corticosterone dose, respectively [43, 47].
GHRH KO mice is a useful model to analyze anti-depressant roles of peptides as TRH since those animals present less immobility time in the FST and TST along with high hypothalamic TRH mRNA levels [30]. Also, KO animals for TRH-R1 [32] or TRH-R2 [33] support the role of limbic TRH in mood regulation since both transgenic mice display depressive-like behaviors.
Female rats in proestrus and estrus present low depression parameters due to the characteristic high estrogen serum levels observed in those phases, which are implicated with anti-depressant actions. In those cycle phases, females show decreased content of TRH in various regions of the limbic system, as if the peptide is being released and thus implicated in estrogen-induced depression regulation [29].
The utilization of electroconvulsive shocks in rats as anti-depressant treatment results in elevated TRH mRNA levels in the granular cells of the hippocampal dentate gyrus analyzed by microarrays [48]; in addition, with the same approach, others find high peptide levels in limbic regions, including the Amy and the pyriform cortex besides the Hipp [49, 50].
Olfactory bulbectomy (OBX) is considered a model of depression and is commonly used in rats to evaluate the efficacy of different anti-depressant drugs. Controversies are found regarding TRH changes in these animals: OBX increases hypothalamic TRH content, and when treated with sertraline, a serotonin reuptake inhibitor, such change is reversed, and their locomotor activity is reduced [51]. In contrast, other studies that also use OBX rats find no changes in brain TRHmRNA and no association with depressive-like behaviors [52, 53].
We only found one in vitro report showing that lithium (anti-depressant) treatment in cultured GH3 cells can interfere with the TRH-induced increase in inositol 1,4,5 triphosphate (IP3), which argues against the anti-depressant effect of the peptide [54].
Only two articles use a different approach to elucidate the role of TRH in mood regulation, which is the injection of the peptide or some of its analogs, allowing the observation of depressive-behavioral changes in rodents. By injecting the TRH analog taltirelin in TRH-R1, TRH-R2 or both KO mice and evaluating their immobility time in the TST, the results point out to TRH-R1 activation as needed for TRH effects as anti-depressant [55].
Choi et al. (2015) [45] (already mentioned in the 3.2.1. section) show that the injection of TRH or taltirelin in the BLA induces depression-like behaviors in animals performing the FST, TST, U-field sociability and U-field tests.
An i.p. injection of TRH in rats induces an increased release but not the turnover of noradrenaline in hypothalamic cultured tissue, which supports the anti-depressant role of TRH [56].
We found seven articles that investigated the effect of administering different
drugs known as anti-depressant factors such as ketamine, prazosin, glycogen
synthase kinase 3
TH administration, which also participates in displaying depression symptoms, induces an increment in brain TRH [59]. As TH can only affect TRH transcription specifically in the hypophysiotopic neurons of the PVN, it is interesting that by stimulating a non-genomic mechanism, TRH levels also change in Amy, pyriform cortex and striatum. With acute and chronic valproate administration or after its withdrawal in rats, TRH content increases in the pyriform cortex, Amy and Hipp, the expression levels of TRH receptors in NAc and PFC, and the receptor binding in Amy and PFC [60]. Acute or chronic consumption of lithium in the diet also changes limbic TRH content, decreasing it in the entorhinal cortex but increasing peptide levels in the pyriform cortex and striatum when lithium withdrawal is induced [61].
We selected ten clinical studies, and some suggest that TRH ameliorates depressive symptoms. Postmortem analyses showed decreases in TRH mRNA levels in the PVN when patients had been chronically treated with corticosterone, which, if elevated in serum in a sustained manner, maybe underlying the development of depression [62]. A similarly reduced gene expression of TRH is observed in PVN of patients diagnosed with major depression [63]. Others have not found changes in limbic TRH since postmortem studies of depressed patients show similar peptide levels in the Amy of males and females and similar to those of the control group [64].
When the analysis is performed in the CSF of depressed untreated patients, TRH levels decrease [65] and even older [66]. In contrast, Frye et al. [67] find no correlation between CSF TRH levels and depression but show gender differences since TRH levels are lower in females than males. Importantly, the difference is greater when patients are depressed [67].
Intra-thecal TRH injection in patients with major refractory depression ameliorates their symptoms, reduces their scores in the Hamilton scale in 50% of the sample as well as their suicidal attempts [4]; i.v. administration of TRH during the night is also successful in decreasing depressive symptoms in patients [68]. When the administration of TRH is oral or i.v. and intermittent for 14 days to depressed patients, their encephaloelectrographic pattern is altered along with enhanced interest, desire, and drive for work, food, and sex, which are symptoms of a mitigated depression [69].
We obtained 289 articles related to TRH and feeding. From these, 31 were reviews and thus omitted. After screening the abstracts, 45 papers met the criteria included in our classifications (See Appendix Table 1).
We found five papers analyzing the role of TRH in models of fasting, food restriction and anorexia, three papers delve into the role of TRH in feeding during negative energy balance, and four studies focus on the role of TRH in different approaches that modify feeding such as, genetic or disease models, and by the modulation of the neuronal activity of specific brain regions.
The initial studies of TRH involvement in feeding include research done in the 1980s reporting an increase of a hypothalamic TRH metabolite (cyclo-His-Pro) after fasting, while no changes in TRH levels from this region are observed [70]; starvation causes a decrease in TRH release from the Hyp into the hypophyseal-portal blood [71]. A more recent finding shows that the reduction in PVN TRH mRNA levels after fasting remains low 4 and 12 h after refeeding [72]. A report where both food-restriction and a model of anorexia were analyzed shows the expected decrease in TRH mRNA levels in the PVN due to negative energy balance in food-restricted animals; nevertheless, anorectic rats do not display such adaptive response, and in turn, show an increase in TRH mRNA levels [34]. An additional report reveals that TRH expressed specifically in the anterior subdivision of the PVN possesses the specific role of inhibiting appetite, even in starved rodents in the model of dehydration-induced anorexia [73].
One report [74] shows hibernating ground squirrels have alterations in their hypothalamic transcriptome that prepare them for the winter. One of these alterations is a 55% decrease in their food intake before hibernation, associated with TRH mRNA upregulation [74].
During fasting, the highly abundant and proximity of NPYergic tonic inhibition to TRHergic neurons in the PVN decreases energy expenditure during negative energy balance [75]. Unexpectedly, an excitatory input to orexigenic AgRP neurons arising from a subset of TRHergic cells that coexpress the pituitary adenylate cyclase-activating polypeptide (PACAP) is necessary and sufficient to drive hunger [76].
On the other hand, the spontaneous hypophagia present in Lou/C rats, a strain resistant to diet-induced obesity, is due to high leptin sensitivity, which induces the overexpression of hypothalamic brain-derived neurotrophic factor (BDNF) and TRH [77].
Excitotoxic lesions of the dorsomedial hypothalamus (DMH) uncover another possible role of TRH in feeding, specifically in the circadian aspect of this behavior. Such lesions disrupt the animals’ circadian food intake distribution, possibly mediated by TRH-expressing neurons that project to the lateral hypothalamus, impinging on orexinergic neurons [78]. Recently, with new technologies of neuronal labeling, a report shows that activation of DMH afferents to PVN TRH receptor-expressing neurons, coexpressing other feeding-regulatory peptides such as cholecystokinin (CCK), prodynorphin and gastrin-releasing peptide, inhibits food intake [79].
In a model of diabetic rats, TRH mRNA expression is downregulated in the PVN correlating with hyperglycemia. This change, along with increases in ARC orexigenic neuropeptides that directly decrease TRH expression levels, is responsible for the hyperphagia observed in animals subjected to this model [80].
Mice with a mutation resulting in the loss of function of the glucose transporter 2 (GLUT2) as a receptor for extracellular glucose have alterations in their feeding patterns, characterized by an increase in their meal size. This is related to an altered neuropeptidergic expression in the Hyp, including downregulation of TRH mRNA, whose synthesis is dependent on the GLUT2 detection function, favoring actions of orexigenic peptides and contributing to defective control of food intake in this mouse strain [81].
Finally, we found one in vitro report that provides further support of the role of TRH in feeding: administration of TRH and one of its agonists hinders MCH neuronal activation through an increase in inhibitory GABAergic neurotransmission in the brain slices [82].
Eleven studies investigate the feeding effects of the peripheral or central administration of TRH or its metabolites, supporting its anorectic role even during starvation; moreover, TRH injection directly into the Hyp of rats causes anorexia and sometimes adipsia [7, 83, 84, 85, 86, 87, 88, 89, 90]. Chronic peripheral injection of TRH in obese rats causes an increase in their resting metabolic rate and a decrease in their food intake. Thus weight gain is reduced [91]. TRH administered i.c.v in rodents reverses the feeding-promoting effects of drugs such as diazepam [92].
The anorectic effect of an i.c.v. Administration of TRH is partially reversed in histamine depleted rats, and central injection of TRH increases the turnover of the NT. Histamine-synthetizing neurons express TRH-R2, and thus authors propose a hypothalamic histaminergic mediation of the anorectic actions of TRH [93]. By using a histamine H1 receptor KO mice followed by anti-TRH antibody administration, the anorectic effects of the peptide nesfatin 1 seem to be mediated by histamine and TRH [94].
Recent work has expanded the knowledge of the TRH system beyond the hypothalamic role of energy homeostasis regulation. Through intracisternal or lateral ventricle administration of a TRH analog, the role of brainstem TRH has been described in more detail. Intracisternal TRH administration increases food intake two to three-fold in ad libitum fed rats, while the changes after lateral ventricle administration are insignificant. In contrast to what is reported in Hyp, after fasting, rats display an enhanced TRH and TRH-R1 mRNA expression in the brainstem. Brainstem TRH can stimulate feeding through a vagal-ghrelin pathway and interact with NPY-Y1 receptors [95].
Moreover, the orexigenic response after an intracisternal administration of a TRH analog is reduced in Goto-Kakizaki rats, a model of type 2 diabetes and with an impaired central vagal function. Natural food intake in these rats is also diminished, supporting the role of altered brainstem TRH in preventing further weight gain in obesity and type 2 diabetes [96].
Finally, only two studies related to accumbal TRH participation in feeding. The direct injection of the peptide into the NAc shell decreases feeding motivation by augmenting DA release and turnover in food-restricted rats [8]. In contrast, accumbal injections of TRH can reduce palatable food intake in stressed animals while increasing serum glucocorticoid (GC) levels when GC signaling is blocked in the NAc of stressed animals, palatable food intake decreases and TRH expression increases. Overall, these results suggest that accumbal TRH is downstream of GCs activity and its effects on palatable food intake [97].
We found 11 studies related to the administration of hormones that regulate energy homeostasis, signaling mediators or receptor antagonists that modulate TRH levels of expression in different brain regions with consequent changes in feeding.
Altering TRHergic signaling through an intracisternal administration of an antisense ODN directed against TRH-R1, the cephalic phase of acid secretion decreases during sham-feeding. Authors propose that cholinergic activation is mediated by medullary TRH [98].
As mentioned in TRH and anxiety section, the i.p. injection of PDE7B inhibitor affects feeding and anxiety depending on the administered doses. While a lower concentration (0.2 mg) of PDE7B inhibitor increases PVN TRH expression and decreases feeding (without affecting anxiety-like behavior), a higher dose enhances accumbal TRH mRNA. It induces anxiolysis, with no changes in food intake [42].
Feeding related hormones such as ghrelin or leptin, when i.p. administered, have opposing effects on TRH levels in several brain regions, provoking an increase and decrease, respectively [57, 99]. Conversely, intra-VMH leptin administration increased TRH mRNA expression and other anorectic peptides in the arcuate-median eminence complex; this correlates with decreased food intake and is supposed to be mediated through a different pathway than when leptin is i.c.v. Administered [100]. I.p. co-administration of CCK and leptin can reduce food intake in rats, which is reversed by the i.c.v. Injection of antibodies against TRH and cocaine- and amphetamine-regulated transcript (CART) [101].
Secretin administration to mice inhibits food intake while increasing the
expression of TRH and the melanocortin four receptor (MC4R) in the PVN,
supporting the interaction between TRHergic and melanocortinergic systems [102].
Moreover, suppose TRH expression is blocked using an antisense ODN. In that case,
the anorectic effect of
Two papers have analyzed early-life exposure to some chemicals in TRH and feeding in rats: fetal exposure to GCs causes a decrease in TRH immunoreactivity in the yuxtaparaventricular region of the lateral hypothalamus, which is associated with increased food intake in adult offspring from both sexes [104]. Also, exposure to tobacco smoke during lactation provokes a decrease in TRH content in the PVN, related to hyperphagia during adulthood [105].
The few studies evaluating TRH involvement in eating disorders only report an impaired response of TSH in the TRH-stimulation test and thus were omitted from the present review. Analysis of genetic variants in extremely obese patients revealed six novel and rare missense variants, including one in the hypothalamic TRH gene, which may probably damage the protein function [106].
This systematic review aimed to investigate the role of TRH in mood and eating regulation. In general, we found that most of the selected literature supports that the amygdalar and accumbal TRHergic systems are inversely associated with anxiety behavior. However, the study of the involvement of NAc is at early stages. Also, TRH content or mRNA expression changes in limbic regions of animals subjected to almost all the depression models. However, the direction of the changes is not always congruent with its proposed role as an anti-depressant. Nevertheless, the TRHergic system in limbic regions is consistently modified and unequivocally involved in mood regulation. Regarding feeding research, most of the studies describe the role of hypothalamic TRH in regulating this behavior as an anorectic peptide.
Studies in humans have contributed to identifying a correlation between CSF TRH concentration changes and the prevalence of mood disorders. Not all the studies included in the present review support that correlation, but the diagnosis of patients with or with no comorbidities, the size of the sample, or even the moment of the day when TRH is measured is not similar between reports, and all those aspects may influence the disparity of the results. Interestingly, when postmortem studies have been performed in diagnosed patients, changes in TRH mRNA levels are still found in specific limbic regions, supporting its involvement with mood disorders [44, 62, 63]. Regarding eating disorders, only one report is associated rare missense variant in the TRH gene with obesity [106].
The use of animal models has helped to understand the role of TRH in mood and feeding independently of its neuroendocrine function. In this regard, the studies using KO mice for TRH receptors have shown that the extrahypothalamic TRH/TRH-R2 system regulates mood, with no interference of thyroid hormones’ functions [107]. Interestingly, changes in TRH content and expression in limbic areas of rodents, such as Hyp, Amy, NAc and Hipp, when subjected to different paradigms of depression, anxiety, anorexia or obesity, support peptide’s involvement in mood eating alterations. It should be noted that the changes described regarding the TRHergic system are not always consistent with its main proposed role; this is explained in some reports by the lack of simultaneous synthesis, cleavage or release processes of the peptide or the route of drug administration.
Most of the studies analyzed to describe the role of TRH from the Hyp in the
regulation of feeding. Hyp TRH is not only involved in energy homeostasis through
its role as the main controller of the HPT axis, but it also regulates feeding
through its widespread efferent connections to several brain areas such as other
hypothalamic nuclei, septal area, Amy and Hipp that in concert control several
aspects of feeding behavior [107]. Hypothalamic TRH neurons receive direct inputs
from neuropeptides related to food intake regulation, supporting its role as a
central regulator of energy homeostasis [108, 109]. Moreover, hypothalamic
TRHergic neurons coexpress feeding regulatory peptides and receptors such as
nesfatin-1, CART, urocortin 3,
It is important to clarify that no antagonists of TRH have been obtained or commercialized up to date. Thus different approaches have been made to overcome this difficulty and analyze its role in the mentioned alterations. Therefore, most pharmacological studies describe only the effects of TRH administration or its analogs. To block the TRHergic pathway for evaluating behavioral changes, it has been used the injection of siRNAs or antisense ODNs directed against the peptide or its receptors or the administration of drugs that interfere with intracellular signaling of TRH receptors. Even with the diversity of approaches, the involvement of TRH in regulating mood processes is supported, although some discrepancies have been described.
Specifically, the TRHergic system of the Amy has been a frequent target for studying the peptide participation in regulating anxiety because of the involvement of this region in fear and anxious responses of animals. The inverse association found between Amy TRH expression and anxiety supports this region’s anxiolytic function of TRHergic neurons. Given that TRH expression changes by administering anti-depressant or anxiolytic drugs, TRH may be modulating the effects of drugs on different NT systems. Both TRH receptors in other limbic regions, such as the NAc, support afferent connections of TRHergic neurons from brain areas such as Amy and peptide’s modulation of accumbal-regulating behaviors such as locomotion hedonic feeding, anxiety, depression, and drug-seeking conducts.
We found only a few reports where eating and mood disorders are studied in relation to TRHergic system changes in limbic regions. However, such association cannot be discarded, and the studies we included in this review support this relation. The different effects on mood that result from modulating TRH expression may be beneficial to treat patients diagnosed with eating disorders.
The biological functions of TRH are diverse. By controlling the thyroid axis, it maintains energy homeostasis prioritizing its availability or storage depending on the changing needs of individuals. In the brain, TRH can stimulate or inhibit other regions where the peptide-synthesizing neurons project and mediate the effects of different neurotransmitters by acting on presynaptic cells. Diverse studies have proposed TRH with effects in arousal, locomotion, memory, and learning processes, but here we show evidence of its role in mood and feeding regulation. Given that it can mediate cellular stress responses in the limbic system, TRH may participate in various aspects of mood and feeding disorders. Therefore, results are interesting to delineate the specific circuitry underlying TRH effects on mood disorders to find new therapeutic strategies to cope with these diseases.
There is little information regarding the interconnections of TRHergic cells from different brain regions involved in a unique mood or feeding disorder. Describing afferent projections of TRH-synthesizing neurons from the Amy, NAc, Hipp, and PFC, the postsynaptic cells affected, and those incoming cells efferent to TRH pathways would help to understand and regulate its various actions and participation in mood and eating regulation.
We consider that some specific approaches that can be pursued are the following:
To design molecules to specifically block TRH effects as antagonists, which are not commercially available. This is a pending strategy that will contribute to identifying peptide involvement in the functions above and pathologies.
The activation or inhibition of specific TRHergic cells with optogenetics will help dissect if TRH from limbic regions is underlying the feeding alterations observed in animal models of mood disorders.
To identify TRH signaling components and manipulate gene expression in combination with animal models of feeding disorders (e.g., activity-based anorexia model).
To extend studies using female animals based on their emotional profile, analyze limbic TRH effects on mood and feeding regulation, and compare them with male responses.
PdG conceived the issue; EAS, CGL, PSC and PdG performed the acquisition, analysis, and interpretation of data, and wrote the paper. EAS, CGL and PSC designed the figure and table.
Not applicable.
We thank Isabel Amaya and Gilberto Matamoros from the INPRFM for their contribution and technical assistance.
This study was supported by grants from the CONACyT (45131 and 128316 to PdG).
The authors declare no conflict of interest.
See Table 1 (Ref. [4, 6, 7, 8, 29, 30, 31, 32, 33, 34, 35, 36, 37, 38, 39, 40, 41, 42, 43, 44, 45, 46, 47, 48, 49, 50, 51, 52, 53, 54, 55, 56, 57, 58, 59, 60, 61, 62, 63, 64, 65, 66, 67, 68, 69, 70, 71, 72, 73, 74, 77, 78, 80, 81, 82, 83, 84, 85, 86, 87, 88, 90, 91, 92, 93, 94, 95, 96, 97, 98, 99, 100, 101, 102, 103, 104, 105, 106]).
Report | TRH changes | Region analyzed | Treatment or animal model | Behavioral changes |
(1) TRHergic system in animal models | ||||
Anxiety | ||||
Pekary & Sattin 2014* [29] | Hyp, Amy, Hipp, NAc | Proestrus adult female Sprague-Dawley (SD) rats | N/A | |
Leone et al., 2014* [30] | Hyp | GHRH KO mice | ||
Gutierrez-Mariscal et al., 2008, 2012 [6, 31] | Amy | Wistar and WKY rats exposed to EPM and DBT | N/A | |
Zeng et al., 2007* [32] | N/A | N/A | TRH-R1 KO mice | |
Sun et al., 2009* [33] | N/A | N/A | TRH-R2 KO mice | |
Jaimes-Hoy et al., 2008 [34] | PVN | Dehydration-induced anorexic female rats | ||
Depression | ||||
Kim et al., 2017 [46] | BLA | Chronic stress/Interleukin-18 KO mice | ||
Choi et al., 2015 [45] | BLA | Chronic stress and TRH, TRH-R1 silencing expression with siRNAs | ||
Shukla et al., 2010 [41] | Amy | Gestational T |
No changes in depression parameters | |
Pekary et al., 2008 [47] | Amy, PFC | Chronic corticosterone administration | N/A | |
Pekary et al., 2006 [43] | Amy, Hipp, Striatum, entorhinal cortex | Acute corticosterone administration SD rats | N/A | |
Ploski et al., 2006 [48] | DG | Electroconvulsive shocks | N/A | |
Bissette et al., 2001 [51] | Hyp | Olfactory bulbectomy | N/A | |
Pekary et al., 2000 [50] | Amy, piriform cortex, Hipp | Electroconvulsive shocks | N/A | |
Holmes et al., 1999 [52] | No changes TRH mRNA levels | Ventral striatum | Olfactory bulbectomy | N/A |
Holmes et al., 1998 [53] | No changes TRH mRNA levels | Reticular thalamic nucleus, PVN | Olfactory bulbectomy | N/A |
Lazarus et al., 1998 [54] | Blocking of the TRH intracellular signaling | GH3 pituitary cells | In vitro effect of lithium | N/A |
Kubek et al., 1985 [49] | Amy, piriform cortex, Hipp | Electroconvulsive shocks | N/A | |
Feeding | ||||
Sonoda et al., 2019 [80] | PVN | Diabetic rats | Hyperphagia | |
Schwartz et al., 2015 [74] | Hyp | Hibernating ground squirrels | Hypophagia | |
Veyrat-Durebex et al., 2013 [77] | Hyp | Lou/C rats (DIO resistant strain) | Hypophagia | |
Alvarez-Salas et al., 2012 [73] | PVN | Food restriction/dehydration-induced anorexia | N/A | |
Zhang & van den Pol 2012 [82] | Administration of TRH in vitro | Brain slices | Administration of TRH in vitro hinders MCH neuronal activation | N/A |
Stolarczyk et al., 2010 [81] | Hyp | Mutation: loss of functions GLUT2 as a glucose receptor | Increased meal size | |
Jaimes-Hoy et al., 2008 [34] | Hyp | Food restriction/dehydration-induced anorexia | N/A | |
Sanchez et al., 2008 [72] | Hyp | Fasting/refeeding | N/A | |
Chou et al., 2003 [78] | Inhibition of TRH neurons | DMH/LH | Excitotoxic lesions of DMH | Disruption of circadian distribution of food intake |
Rondeel et al., 1992 [71] | Hyp | Starvation | N/A | |
Mori et al., 1983 [70] | Hyp | Fasting | N/A | |
(2) Exogenous TRH administration and changes in behavior | ||||
Anxiety | ||||
Gutierrez-Mariscal et al., 2008 [31] | N/A | N/A | I.c.v. TRH administration to Wistar rats | |
Siqueira et al., 2010 [36] | N/A | N/A | I.p. TRH administration to Wistar rats | No effect on anxiety in EPM |
Gargiulo & Donoso 1996 [35] | N/A | N/A | I.c.v. TRH administration to Holtzman rats | No effect on anxiety in EPM |
Depression | ||||
Choi et al., 2015 [45] | BLA | BLA TRH administration and analog taltirelin in C57BL/6 mice | ||
Thirunarayanan et al., 2013 [55] | No changes on TRH-R1 and TRH-R2 mRNA expression | All brain | I.p. analog taltirelin to TRH-R1, TRH-R2 or both KO mice | |
Horst et al., 1974 [56] | N/A | Hyp | I.p. TRH administration to rats | N/A |
Feeding | ||||
Alvarez-Salas et al., 2019 [97] | N/A | N/A | Intra-NAc injection of TRH to Wistar rats | Reduce palatable food intake |
Puga et al., 2016 [8] | N/A | N/A | Intra-NAc injection of TRH to food-restricted Wistar rats | Reduce food intake, low motivation to eat |
Zhao et al., 2013 [96] | N/A | N/A | Intracisternal TRH to type 2 diabetes rat model | No increase in food intake |
Schuler et al., 2007 [90] | N/A | N/A | I.c.v./i.p TRH to Siberian hamsters | Both routes TRH decrease food intake |
Gotoh et al., 2007 [93] | N/A | N/A | I.c.v. TRH administration to SD fasted rats | Suppress food intake, mediated by Hyp histamine |
Ao et al., 2006 [95] | N/A | N/A | Intracisternal administration of TRH analog to SD rats | Stimulated food intake in freely fed rats |
Al Arabi et al., 2006 [91] | N/A | N/A | Chronic peripheral infusion of TRH to Zucker fa/fa rats | Increases resting metabolic rate and inhibits food intake |
Choi et al., 2002 [7] | N/A | N/A | Acute and chronic s.c. TRH injection to ad libitum fed SD rats | Acutely reduced food and water intake chronically only increase water intake. |
Bowden et al., 1988 [88] | N/A | N/A | I.c.v./i.p TRH/ TRH metabolite administration to sated/fasted SD rats | TRH i.c.v./i.p. decrease food intake in food restricted rats, i.c.v. TRH metabolite increases spontaneous feeding |
González et al., 1984 [92] | N/A | N/A | I.c.v. TRH administration + oral diazepam to Wistar rats | Decreases diazepam-induced hyperphagia |
Lin et al., 1983 [87] | N/A | N/A | I.c.v. TRH administration to fasted Sprague-Dawley rats | Food but not water intake is reduced |
Luttinger et al., 1982 [86] | N/A | N/A | I.c.v. TRH administration to fasted Sprague-Dawley rats | Suppress food intake |
Suzuki et al., 1982 [85] | N/A | N/A | I.c.v. and 6 different brain regions TRH administration to fasted Wistar rats | Suppress food and water intake in a dose-dependent manner, mostly in the medial hypothalamus |
Vogel et al., 1979 [84] | N/A | N/A | I.p. and i.c.v. TRH administration to SD rats | Decreases food reinforcement, both i.p. and i.c.v. decreases food and water intake |
Vijayan & McCann 1977 [83] | N/A | N/A | I.c.v. TRH administration to fasted SD rats | Suppress food and water intake in a dose-dependent manner |
(3) Exogenous drug administration and changes in endogenous central TRHergic system | ||||
Anxiety | ||||
Trujillo et al., 2020 [40] | No changes on TRH mRNA | PVN | Neonatal serotonin depletion in rats | |
Valdés-Moreno et al., 2017 [42] | NAc | Accumbal and high i.p. doses administration of PDE7 inhibitor | ||
Pekary et al., 2015 [37] | Hyp, NAc, PFC, Amy | Ketamine i.p. injected SD rats | N/A | |
Sattin et al., 2011 [38] | Amy, NAc, striatum | Prazosin i.p. injected SD and Lewis rats | N/A | |
Shukla et al., 2010* [41] | Amy | Gestational T4 replacement in WKY rats | ||
Pekary et al., 2006 * [43] | Amy, Hipp, Striatum, entorhinal cortex | Acute corticosterone administration SD rats | N/A | |
Sharif & Burt 1984 [39] | Amy | Inhibition of TRH receptor binding by benzodiazepines in central tissue of SD rats | N/A | |
Depression | ||||
Pekary et al., 2010 [99] | Amy | GSKI i.p. injected SD rats | N/A | |
Pekary et al., 2008 [47] | Amy, PFC | Chronic corticosterone administration | N/A | |
Sattin et al., 2008 [58] | NAc | Escitalopram i.p., injected SD rats | N/A | |
Pekary et al., 2006 [59] | No changes on TRH content | Amy, NAc | PTU or T4 i.p. injected SD rats | N/A |
Pekary et al., 2004 [60] | NAc, PFC | Valproate i.p. injected WKY rats | N/A | |
Sattin et al., 2002 [61] | NAc, Amy | Lithium oral administration Wistar and WKY rats | N/A | |
Sattin et al., 2002 [61] | Hyp, striatum | Lithium oral administration SD rats | N/A | |
Lazarus et al., 1998 [54] | Blocking of the TRH intracellular signaling | GH3 pituitary cells | In vitro effect of lithium | N/A |
Feeding | ||||
Alvarez-Salas et al., 2020 [103] | N/A | NAc | asODN fasted rats | Anorectic effects of a-MSH |
Pexioto et al., 2019 [105] | PVN | Tobacco smoke exposure during lactation Wistar rats | Adult hyperphagia | |
Valdés-Moreno et al., 2017 [42] | PVN | Low i.p. dose administration of PDE7 inhibitor | ||
Akieda-Asai et al., 2014 [101] | Hyp | CCK and leptin i.p. injected Wistar rats | ||
Gotoh et al., 2013 [94] | Hyp | Nesfatin 1 i.p administration | Hypophagia | |
Carbone et al., 2012 [104] | Lateral Hyp | Fetal exposure to glucocorticoids in rats | Hyperphagia | |
Pekary & Sattin 2012 [57] | Hyp, PFC, Hipp, Amy, NAc, striatum | Ghrelin i.p. injected SD rats | N/A | |
Cheng et al., 2011 [102] | PVN | Secretin i.p.injected wild-type mice | Inhibition of food intake | |
Pekary et al., 2010 [99] | NAc, Hipp, Amy, striatum PFC | Leptin i.p. injected SD rats | N/A | |
Ambati et al., 2009 [100] | ARC-ME | Leptin intraVMH injected SD rats | ||
Martínez et al., 2002 [98] | N/A | N/A | asODN TRH-R1 intracisternal-injected SD rats | |
(4) Clinical studies | ||||
Anxiety | ||||
Fossey et al., 1993 [44] | No changes on TRH content | CSF | Anxiety patients | N/A |
Depression | ||||
Alkemade et al., 2005 [62] | PVN | Postmortem tissue of corticosterone-treated patients | N/A | |
Alkemade et al., 2003 [63] | PVN | Postmortem tissue of diagnosed depressed patients | N/A | |
Sharma et al., 2001 [65] | CSF | Untreated depressed patients | ||
Szuba et al., 2000 [68] | N/A | N/A | I.v. TRH injection depressed diagnosed patients | |
Frye et al., 1999 [67] | CSF | Depressive patients | N/A | |
Marangell et al., 1997 [4] | N/A | Intrathecal | TRH injection to major refractory depression diagnosed patients | |
Banki 1992 [66] | CSF | Depressed elder patients | N/A | |
Biggins et al., 1983 [64] | No changes on TRH content | Amy | Postmortem depressive patients | N/A |
Itil et al., 1975 [69] | N/A | Brain function | Oral or i.v. TRH administration to depressed diagnosed patients | |
Feeding | ||||
Mariman et al., 2015 [106] | Obesity-associated SNP TRH gene | Hyp | Obese patients | N/A |
* Reports that include data in both anxiety and depression sections. Abbreviations: Amy, amygdala; aPVN, anterior hypothalamic paraventricular nucleus; asODN, antisense oligonucleotide; ARC-ME, arcuate median eminence complex; BLA, basolateral amygdala; BPRS, Brief Psychiatric Rating Scale; CSF, cerebral spinal fluid; CART, cocaine and amphetamine-regulated transcript; CCK, cholecystokinin; DBT, defensive behavior test; DG, dentate gyrus; DMH, dorsomedial hypothalamus; EPM, elevated plus maze; FST, forced swimming test; GSKI, glycogen synthase kinase-3 b inhibitor; GHRH, growth hormone-releasing hormone; Hipp, hippocampus; Hyp, hypothalamus; PVN, hypothalamic paraventricular nucleus; IR, immunoreactivity; i.c.v., intracerebroventricular; i.p., intraperitoneal; KO, knockout; LH, lateral hypothalamic nucleus; mPVN, medial hypothalamic paraventricular nucleus; MCH, melanin-concentrating hormone; N/A, not applicable; NIHT, novelty-induced hypophagia test; NAc, nucleus accumbens; OFT, open field test; PDE7, phosphodiesterase 7; PTU, propylthiouracil; SD, Sprague-Dawley; TST, tail suspension test; TRH, thyrotropin-releasing hormone; TRH-R1, thyrotropin-releasing hormone receptor 1; TRH-R2, thyrotropin-releasing hormone receptor 2; VMH, ventromedial hypothalamic nucleus; WKY, Wistar Kyoto. |