¶Dedicated to the memory of Professor Marcello Brunelli.
Invertebrate animal models show simple behaviors supported by neural circuits easily accessible for experimentation and yet complex enough to provide necessary information on the cellular and molecular mechanisms that govern the vertebrate nervous system’s function. The mechanisms underlying simple forms of learning have been extensively studied in the marine gastropod Aplysia californica, in which elementary non-associative learning of the behavioral habituation and sensitization type has been studied using the gill withdrawal reflex. A strong stimulus applied to the neck or tail improves the reflex response through heterosynaptic facilitation. The neurotransmitter serotonin is involved in both behavioral sensitization and dishabituation by acting through the second messenger cyclic adenosine monophosphate, protein kinase A, the phosphorylation of a K
Growing evidence on elementary forms of learning processes in selected animal models has highlighted some mechanisms representing the basic cellular and molecular principles underlying plastic changes. Learning is defined as the ability to modify the behavior to experience and environmental changes, and memory is the retention of that modification over time. We define explicit memory that includes people and things that can explain conscious awareness, and implicit memory includes procedures that do not require conscious awareness. In turn, implicit memory comprises non-associative and associative learning, which are the simpler forms of learning.
To investigate the cellular and molecular mechanisms at the basis of learning, a reductionist approach has been fundamental, identifying a simple animal model with simple behaviors, such as reflex, which undergoes simple forms of non-associative learning, such as habituation, dishabituation and sensitization (Brunelli et al., 1997). The simplicity of neural circuits of invertebrate has facilitated the knowledge of neural mechanisms underlying learning. Invertebrate animal models show simple behaviors supported by neural circuits that are easily accessible for experimentation yet still complex enough to highlight the cellular and molecular mechanisms that govern the more complex nervous system’s function in vertebrates. The invertebrate model organism that has provided information for studying the molecular mechanisms of memory more than any other in the last decades is the attractive model of marine gastropod Aplysia californica (Kandel, 2001). Do the mechanisms of implicit memory observe in Aplysia found a parallel in other animals? For example, research on fear learning conducted on the fruit fly Drosophila indicated that implicit memory’s molecular mechanisms have strong similarities to those of Aplysia (Kandel, 2012).
The purpose of this brief report is to discuss the best known and studied invertebrate model for the mechanisms underlying learning, which is that of Aplysia, and then a second animal model represented by the induction model to swim of the leech Hirudo medicinalis.
Aplysia has a straightforward ganglionic nervous system that has proved to be an ideal model for studying the mechanisms underlying non-associative learning (Kandel, 2001). Aplysia possesses a group of simple reflexes that can be modified by different forms of learning. A paradigmatic example is the gill withdrawal reflex. A weak tactile stimulus applied to the siphon causes a rapid withdrawal of the gill and the siphon into the mantle cavity. The neural circuit that mediates this reflex has been thoroughly studied. The circuit has a monosynaptic component in which the sensory neurons innervating the siphon make direct connections with the motor neurons that move the gill (Byrne and Hawkins, 2015). The tactile stimulus on the siphon excites the sensitive mechanoreceptors that innervate the siphon. The neurotransmitter glutamate is released from the sensory endings and gives rise to excitatory postsynaptic potentials (EPSPs) in motor cells. The EPSPs undergo summation on motor cells that respond by producing the retraction movement of the gill. This reflex undergoes various forms of non-associative learning, such as habituation, dishabituation and sensitization, the latter representing a form of learned fear.
Habituation is a progressive reduction of the reflex response to a weak, repeated stimulus and is the simplest form of non-associative learning. Habituation is an adaptive phenomenon and works as a selective filter that allows animals to ignore irrelevant stimuli (Castellucci et al., 1970). The mechanism underlying habituation is homosynaptic depression (Bailey and Chen, 1988; Glanzman, 2009).
Sensitization is a form of learning in which repeated exposure to a noxious stimulus induces an increase in reflex reactivity (Castellucci et al., 1989). In Aplysia, a shocking stimulus applied to the tail frightens the animal, so much so that the same weak tactile stimulus that had induced a moderate defensive retraction reflex of the gill at rest now produces a much more vigorous and lasting reflex action of withdrawal. This behavior is defined as dishabituation if it follows a habituation session.
It is well-known that the retention of information has two components: a short-term (ST) learning process and a long-term (LT) learning form. The duration of the memory for the noxious event changes due to the number of training trials. Repetition of an experience transforms ST memory into LT memory. A single tail shock can induce ST sensitization that lasts for minutes, while a series of time-spaced shocks produces LT sensitization that lasts for days or weeks or longer.
The gill retraction reflex in Aplysia showed that synaptic plasticity (neurons’ ability to modulate strength and structure of synapses) is a key mechanism contributing to learning and memory storage. These forms of short- and long-term synaptic plasticity underlying learning require distinct molecular requirements. In particular, in ST, it is firmly established that noxious sensitization stimulus produces heterosynaptic facilitation of the monosynaptic sensorimotor connection through activation of serotonergic interneurons synapses with sensory neurons of the siphon (Antonov et al., 1999; Brunelli et al., 1976). The neurotransmitter serotonin (5-hydroxytryptamine, 5-HT) is released from modulatory interneurons on presynaptic receptors to induce an increase in neurotransmitter release glutamate at sensory-motor neuron synapses. 5-HT is essential for inducing behavioral sensitization (Glanzman et al., 1989).
Furthermore, Aplysia’s serotonergic system exhibits various characteristics common to mammals, including arousal, feeding, and locomotion functions (Marinesco et al., 2004). 5-HT facilitates sensory-motor synapse through the activation of a Gs-coupled receptor (Brunelli et al., 1976). In particular, 5-HT activates an adenylate cyclase into the terminals of sensory neurons, involving cyclic adenosine monophosphate (cAMP), that induces activation of protein kinase A (PKA) and phosphorylation of pre-existing proteins, decrease of K
So, the ST sensitization depends on the covalent modifications of pre-existing proteins through PKA activity. G protein-coupled 5-HT receptor activation stimulates the formation of the second messenger inositol 1,4,5-triphosphate (IP
What are the events that are involved in the LT learning process? The most suggestive evidence is the idea that LT memory requires transcription and translation processes. Modifications in gene expression attended LT memories. In particular, many training trials or repeated applications of 5-HT induce a prolonged activation of PKA, allowing the catalytic subunit to transfer to the nucleus of sensory neurons. In the nucleus, PKA phosphorylates the transcriptional activator cAMP-responsive element-binding protein (CREB-1) (Si and Kandel, 2016). CREB-1 binds to a regulatory region of genes called CRE (cAMP-responsive element). The activation of transcription by PKA also depends on its ability to activate the mitogen-activated protein kinase (MAPK) pathway. The phosphorylation by MAPK from the transcription factor CREB-2 reduces its inhibitory action on transcription. Thus, the combined effects of CREB-1 activation and reduction of CREB-2 repression result in the expression of a cascade of novel genes important for learning and memory phenomena. CREB-2 is inhibited by an increase of 5-HT in extracellular signal-regulated kinase (ERK). So, LT learning involves both new protein synthesis and CREB-mediated gene expression. In turn, they drive the remodeling of pre-existing synapses and the formation of new synapses. In particular, event transduction involves various feedback pathways and multiple proteins. For example, an increase of ubiquitin hydrolase enzyme determines a persistence of PKA activation, the secretion of neurotrophin, leading to tyrosine kinase autoreceptors’ activation, the activation of peptide sensor and transforming growth factor (TGF)-ß. In turn, this last one activates ERK and induces its translocation to the nucleus in a positive feedback loop (Si and Kandel, 2016).
Interestingly, the persistence of LT sensitization depends on cytoplasmic polyadenylation element-binding protein (CPEB). This protein has prion-like properties because it exists in two different conformations, one dominates, and it is also self-perpetuated. In particular, the self-perpetuating form is increased by 5-HT application, and it is required for the persistence of LT sensitization. So, CPEB plays a crucial role in carrying the protein synthesis required to maintain LT sensitization (Miniaci et al., 2008; Si et al., 2010; Heinrich and Lindquist, 2011). Since LT synaptic plasticity requires gene transcription phenomena and, for this reason, the nucleus, accessible from other synapses of the neuron, raises whether all LT memory changes must necessarily affect the entire cell and if the changes in LT processes can only be restricted to some synapses. In this regard, Frey and Morris (1997) suggested the intriguing hypothesis of synaptic capture, or synaptic tagging, which proposed two possible explanations. First, gene expression products are taken by all synapses but are functionally assumed only into the synapses tagged by previous synaptic activity. The second explanation is that the activated synapses’ gene expression products are targeted only at the synapse tagged by synaptic activity (Redondo and Morris, 2011).
Since mRNAs are found in the cell body, they are likely dormant before reaching the marked synapse. Since mRNAs are distributed to all terminals, the need for local translation of some mRNAs is assumed only in some synapses, suggesting that these mRNAs may be dormant before reaching the translation site (Kandel, 2012) (Fig. 1).
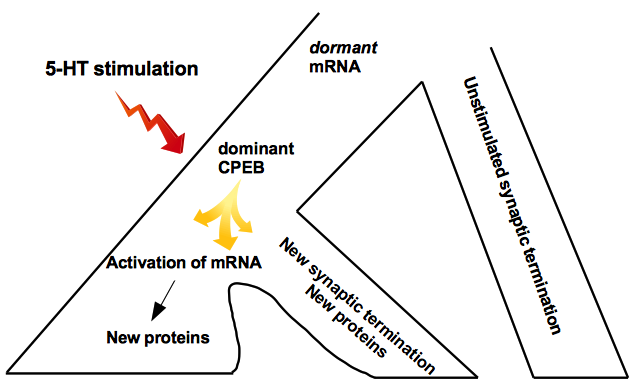
Synaptic capture hypothesis. Scheme of synaptic capture hypothesis for the LT memory mechanism. Locally synthesized proteins are needed to support the growth of new synapses triggered by gene expression. Gene expression products of activated synapses are targeted only at the synapse tagged by synaptic activity.
A series of pieces of evidence supported these hypotheses. CPEB acts as a conformational switch in the brain to enable the stabilization of memory. In 2016 Si and Kandel postulated an intriguing possibility and demonstrated a physiological signal as the synaptic activity, as the neurotransmitter 5-HT could regulate the conversion of CPEB to the multimeric state.
Since these CPEB proteins have prion-like propagation in various animal models, invertebrate and non-invertebrate, Kandel hypothesized that they are not biological anomalies but a ubiquitous and crucial regulatory mechanism (Si and Kandel, 2016; Rayman and Kandel, 2017).
Analyses of non-associative learning in other invertebrate models, as the leech Hirudo medicinalis, have confirmed and extended evidence of Aplysia (Zaccardi et al., 2001). The leech was used to investigate the cellular mechanism underlying elementary forms of learning and provide evidence for non-associative learning processes (Federighi et al., 2013; Macchi et al., 2008). Leech behavioral repertoire was provided, such as the shortening reflex and swimming induction triggered by brush strokes (Zaccardi et al., 2004; Ristori et al., 2006).
This swims by undulating its body on the dorsal-ventral plane and generating a wave along the body regarding the leech’s swimming behavior. In the leech, the neural circuit underlying swimming comprises various components that transduce the skin’s mechanical stimulation into the motor neuron’s rhythmic activity (Brodfuehrer and Thorogood, 2001). The leech CNS comprises 21 segmental ganglia, six fused ganglia in the head ganglion and seven fused ganglia in the tail ganglion. In each segmental ganglion, three types of sensory neurons have been identified, tactile (T cells), pressure-sensitive (P cells), and nociceptive (N cells). Intense tactile stimulation on the body wall induces sensory cells, beginning a cascade of interactions between various neuronal populations culminating in swimming activity. The swim-generating network is an ensemble of neurons, including a central pattern generator and oscillator. It includes five different functional classes of established cells (mechanosensory, trigger, gating, oscillator and motor neurons) that convert mechanosensory input into the swim motor program. Mechanosensory neurons interact with swim trigger interneuron cells connected with swim gating interneurons, which, in turn, interact with oscillator cells that, finally, control dorsal and ventral motor neurons (Brodfuehrer and Thorogood, 2001).
Hirudo’s locomotor behavior is fascinating, as it was found that a higher-order decision-command neuron is required for intersegmental coordination of swimming behavior, providing a model for understanding the network that governs behavior (Wagenaar, 2015). In leeches with the head ganglion disconnected from the first segmental ganglion, light electrical stimuli applied to the skin causes swimming episodes with constant latency (Zaccardi et al., 2001, 2004). It has been observed that repetitive stimulation at a constant inter-trial interval (ITI) induces an increase in swimming latency according to the definition of habituation. A training session of 15 stimuli with ITI of 1 min produces an 80% reduction in the swimming response, which remains for 20-30 min and is not subject to the activation of the cAMP pathway (Zaccardi et al., 2001, 2004; Ristori et al., 2006; Macchi et al., 2008; Traina and Scuri, 2017).
At the cellular level, mild electrical stimulation of the skin activates T neurons explicitly and evokes rhythmic activity in the motor nerves (Scuri et al., 2002; Kristan et al., 2005; Debski and Friesen, 1987). In nearly-intact preparations, low-frequency repetitive application of weak stimuli to the caudal portion of the body induces the habituation of swimming induction, while brush strokes on the dorsal skin lead to sensitization or dishabituation if the leech was previously habituated. The leech’s serotonergic system resides in a limited number of neurons that play crucial roles in various behaviors, such as feeding and swimming. A typical ganglion has six serotonergic cells, including a pair of large Retzius cells and two swim circuit interneurons. Also, in the leech model, 5HT has a fundamental role in behavioral dishabituation and sensitization (Burrell et al., 2001). It has also been reported that 5-HT acts via cAMP (Zaccardi et al., 2004; Traina et al., 2013).
In the leech, are the mechanisms underlying behavioral habituation and dishabituation of swimming induction similar to those described in Aplysia, or are additional mechanisms present? The leech’s nervous system’s first neuronal station consists of the mechanosensory neurons located in each ganglion. A train of action potentials causes in T cells long-lasting after-hyperpolarization (AHP), which in these cells depends both on the Na
Although the phenomenon of habituation is ubiquitous throughout the animal kingdom, the underlying mechanisms remain challenging to identify, particularly when compared with other forms of learning (Christoffersen, 1997; Thompson and Spencer, 1966). A study reported evidence on molecular mechanisms underlying behavioral habituation on swimming in the leech (Zaccardi et al., 2012). Both the entry of Ca
At the cellular level, 5-HT induced a reduction of the AHP, which accompanies bursting in T neurons, affecting the electrical activity and modulating synaptic efficacy between T cells and their followers (Brunelli et al., 1997).
Knowledge of cellular mechanisms provides the framework for a comprehensive understanding of the molecular basis of behavioral habituation. This will allow us to examine behavioral habituation mechanisms with other forms of non-associative learning in leech and other animal models. The increase in latency of swimming induction observed in ST habituation is supported by the same biochemical cascade involved in the activity-dependent increase in AHP exhibited by T neurons during low-frequency stimulation (Scuri et al., 2002). In particular, in sensory T cells, the chain of intracellular events that link repetitive neuronal activity to changes in AHP amplitude requires an increase in intracellular Ca
Various experiments on LT habituation and sensitization in the leech swimming behavioral model have been performed to investigate the leech’s mechanisms underlying LT memory. By applying a session of light stimuli every day, LT habituation was induced after 5- 7 days; similarly, by applying every day a noxious stimulus, LT sensitization was induced. Protein synthesis inhibitors (cycloheximide) and mRNA blocking agents (such as actinomycin D) prevent LT facilitation of swimming induction without affect ST memory (Traina and Scuri, 2015). Therefore, even in this animal model, LT memory requires qualitative and quantitative gene expression and protein synthesis modifications.
Cellular studies of simple animal models’ simple behaviors have provided evidence that synaptic connections between neurons are not immutable but can be modified with learning. The reductionist analysis of neuronal plasticity and simple memory in the invertebrate provides us with the molecular and cellular building blocks and operational rules underlying the exploration of more complex memory systems. It has been confirmed that the same synaptic facilitation phenomena are present in the vertebrate. Non-associative learning appears biologically essential and evolutionarily adaptable. Many nervous system properties are highly conserved. The research using invertebrate models has made an important contribution to understanding the fundamental neuroscience that translates to understanding the human condition. The most significant studies conducted in the giant squid axon have given way to understanding ion channels and their regulation of neuron excitability and synaptic transmission (Hodgkin, 1964). Also, the study on invertebrates is incredibly convenient for the simplicity of the model and the possibility of manipulating the genome. Learning and memory are ubiquitous processes induced through cellular and molecular mechanisms whose alphabetic keys are common to all living beings. These keys are identifiable in the chain of events that bind neurotransmitters and trigger peptides: activation of intracellular messengers, phosphorylation of proteins, a variation of synaptic functions. Crucial was the discovery that the regulatory protein CREB is an important component in the transition from ST to LT memory in many forms of learning, from snails to mice, to humans.
On the other hand, the activation of LT memory requires the switching of genes and to do this, the CREB-1 proteins must be activated, and the CREB-2 proteins must be deactivated. Suppressor proteins are presumed to set a relatively high threshold for converting ST memory to LT memory. We only remember certain events and experiences over time. The fact that a gene must be switched on to give rise to LT memory demonstrates that genes also respond to environmental stimuli and learn.
Although there are significant behavioral differences between explicit and implicit memory, some aspects of the storage of the latter in invertebrates have been preserved for millions of years throughout evolution in those mechanisms by which explicit memory is stored in vertebrates and, today, it is evident that several of the most important molecular mechanisms of memory are common to all animals. Activity-dependent forms of synaptic plasticity induce changes in synaptic transmission that modify existing functional connections. These synaptic connectivity changes’ effectiveness and geometry can provide a basis for learning, memory, and other forms of brain plasticity. The alterations in synaptic efficacy depend on transcription phenomena, and this is a general mechanism of implicit memory, also present in vertebrates.
Explicit memory requires attention. Molecular studies on Aplysia and mice have validated the existence of two forms of attention, involuntary and voluntary. In both types of memory, the conversion from ST to LT memory requires the activation of genes while neurotransmitters carry the attentional signal. In both cases, following this signal, genes are activated, proteins are produced and sent to the synapses. In Aplysia, the attentional signal is used involuntarily in the reflex, and 5-HT is involved while dopamine is involved in the mouse’s explicit memory. A prion-like protein identified in Aplysia has also been identified in the vertebrate hippocampus (Theis et al., 2003). As in Aplysia, 5-HT modulates the CPEB protein, so in the mouse, the hippocampus dopamine modulates a protein analogous to CPEB (Si and Kandel, 2016). This evidence has led to the hypothesis that spatial maps become similar when animals’ attention voluntarily uses a signal. In the vertebrate’s hippocampus, dopamine release initiates a self-perpetuating state, resulting in LT storage. The pathways involved in operant appetitive conditioning in Aplysia are also present in vertebrate reward learning in the striatum (Hawkins and Byrne, 2015). Operant conditioning involves dopamine and cAMP/PKA receptors in the nucleus accumbens (Baldwin et al., 2002). Dopamine is required to potentiate corticostriatal synapses in an analog of reward learning (Reynolds et al., 2001). Striatal neurons show an increase in the intrinsic excitability level that depends on the expression of the transcription factor CREB (Dong et al., 2006). Long-term potentiation in mice is nearly identical to the long-term facilitation that contributes to behavioral sensitization in Aplysia (Rogan et al., 2005). Both have a signaling pathway that includes cAMP, PKA and CREB, indicating that they are all part of a single-family of molecular processes, universal across species.
The learning mechanism has evolved to address an adaptive challenge, and thus invertebrate is likely to face the same adaptive challenge as vertebrate.
Activity-dependent changes in synaptic transmission may be implicated in the onset of some pathologies, such as Alzheimer’s disease, and as recent studies have shown (Teich et al., 2015). Strategies targeting these molecular mechanisms could likely lead to the identification of future treatments. Defining the mechanisms underlying learning and memory could explain the mechanisms governing repair and rehabilitation processes and the development of therapeutic approaches. This knowledge allows us to develop an approach that can connect neural systems to complex cognitive functions and study how we perceive and remember complex experiences.
5-HT, 5-hydroxytryptamine (serotonin); AHP, after-hyperpolarization; cAMP, cyclic adenosine monophosphate; CPEB, cytoplasmic polyadenylation element-binding protein; CREB, cAMP-responsive element-binding protein; EPSP, excitatory postsynaptic potential; ERK, extracellular signal-regulated kinase; IP3, inositol 1,4,5-triphosphate; ITI, inter-trial interval; LT, long-term; MAPK, mitogen-activated protein kinase; PKA, protein kinase A; PLC, phospholipase C; ST, short-term; TGF, transforming growth factor.
GT researched and summarized the information and wrote the paper.
Thanks to all the peer reviewers and editors for their opinions and suggestions.
The author declares no conflict of interest.