† These authors contributed equally.
Correlations amongst the rat glutamatergic system, glia, and depression, as well as the underlying mechanism of astrocyte impairment, as a trigger of depression, were investigated. Rats were submitted to different durations of chronic unpredictable mild stress to induce depressive-like behavior and evaluated by weight change, sucrose preference test, open field test, and novelty suppressed feeding test. High-performance liquid chromatography was employed to detect glutamate content of hippocampal protein expression during Western blot and immunofluorescence. Results showed that 21-day chronic unpredictable mild stress was sufficient for inducing significant depressive-like behavior (reduced body weight and sucrose preference, increased feeding, and immobility time) in a model of depression. Chronic unpredictable mild stress increased the level of hippocampal glutamate, while intervention caused a considerable rise in the expression levels of Bax, caspase 3, and calcium/calmodulin-dependent protein kinase II, accompanied by a down-regulated level of B-cell lymphoma-2. Exposure to this stress model reduced hippocampal glutamate ionotropic receptor N-methyl-D-aspartic acid type subunit 2A, neuronal nuclear protein, and glial fibrillary acidic protein expression levels while it raised the level of ionotropic glutamate receptor N-methyl-D-aspartic acid type subunit 2B level. It is concluded that chronic stress induces excessive glutamate release and overstimulation of N-methyl-D-aspartic acid receptors, followed by astrocytic apoptosis. Also, in depression, calcium overload in astrocytes is attributed to an underlying mechanism of astrocyte impairment.
Accumulating studies reveal that the glutamatergic system is implicated in the etiopathology and treatment of depression (Deutschenbaur et al., 2016). For instance, glutamate levels are affected in the plasma (Mauri et al., 1998), cerebrospinal fluid (Frye et al., 2007), and the depressed brain (Hasler et al., 2007; Sanacora et al., 2008) and revealed by magnetic resonance spectroscopy (Yildiz-Yesiloglu and Ankerst, 2006) and post-mortem studies (Hashimoto et al., 2007), thus supporting a relation between glutamate and depression.
The function of glial cell reuse of glutamate via the glutamate/glutamine cycle (Lebon et al., 2002) is particularly related to the primary etiology of mood disorders (Kugaya and Sanacora, 2005). Moreover, glial cell inactivity can result in elevated glutamatergic activation and neural toxicity (Soriano and Hardingham, 2007). Decreased neurogenesis in the hippocampus is considered as a mechanism linked to the pathophysiology of depression (Kim et al., 2007; Santarelli et al., 2003). In particular, a reduction in glial cell number and density is reported by several post-mortem reports (Cotter et al., 2001; Ongur et al., 1998; Rajkowska et al., 1999).
Chronic stress changes hippocampal synaptic plasticity (Pavlides et al., 2002). The NMDA receptor is one of three ionotropic receptors that mediate synaptic plasticity, and it contains six regulatory subunits. Among the subunits, NR2A and NR2B have been the most extensively studied due to their broad expression in the brain (Yashiro and Philpot, 2008). NR2B tends to promote neuronal death (Kohr, 2006), while NR2A is conducive to neuroprotection (Liu et al., 2007). Notably, over-activated extrasynaptic NR2B induces glutamate excitotoxicity and has strong calcium permeability once it is bound by excessive glutamate (Zhang et al., 2013). Calcium influx via NMDA receptors is required for the initiation of synaptic plasticity (Marsden, 2013). It activates calcium/calmodulin-dependent protein kinase Ⅱ (CaMKⅡ) (Byrne et al., 2009; Deutschenbaur et al., 2016; Pepke et al., 2010). The quantity of activated CaMKⅡ is proportional to the total amount of calcium (Li et al., 2012).
Adult male Sprague-Dawley rats (200-220 g) were supplied by the Experimental Animal Research Center, Nanjing, China. They were acclimatized to the new environment (room temperature: 23±2 °C; relative humidity: 45±15%; light condition: light on 8:00 a.m., 12 L : 12 D light/dark cycle) for one week prior to further processing and were housed in sterilized propylene cages containing sterile husk changed daily. Rats were randomly assigned to one of four groups (10 rats per group): control group, 7-day CUMS group, 14-day CUMS group, and 28-day CUMS group. All experimental procedures were approved by the Animal Use and Care Committee in the Animal Research Center of Zhejiang Chinese Medical University (NO. 20170401-02). Both the study protocol and animal care followed the rules of the Animal Use and Care Committee in the Animal Research Center of Zhejiang Chinese Medical University.
Rats were isolated in separate cages and subject to the daily application of various stressors, scheduled at random over seven days. Stressors included water deprivation (24 hours), fasting (24 hours), tail clamping (1 minute), reversal of day/night cycle (12 hours/12 hours), forced swimming (4 ℃ water, five minutes), heat stimulation (45 ℃ water, five minutes), foot shock (50 mV, 10 s/one shock, 30 s duration, × 15), level shaking (10 minutes).
Furthermore, the weight and food consumption of each rat was recorded each week (days 0, 7, 14, 21, 28) for observation of the physiological state during the CUMS procedure.
The apparatus was an 80 cm × 80 cm × 40 cm square iron box placed in a soundproof room. The floor was painted black and separated by white lines into 25 equal squares (each 16 cm × 16 cm). Each rat was placed at the center, then left to freely move for five minutes. The number of line crossings and times of rearing was recorded by a camera. The box was sterilized with 70% ethanol before testing the next rat.
A fast of 24 hours was implemented before the test phase. On the test day, each rat was individually relocated to a plexiglass open field box (76.5 cm × 76.5 cm × 40 cm) that contained a small pallet of food placed at the center. Each rat was put with the same orientation in the same corner of the box. The latency to eating, identified as the rat sitting on its haunches and biting food held with its forepaws, was recorded within the six-minute observation period.
Each rat was trained (adaptation phase) to consume sucrose solution (1% w/v) for three days. No food and water were provided for 24 hours before the test day. On the test day, two bottles were placed in each rat cage: one contained normal drinking water (200 g), the other a 1% sucrose solution (200 g). After 24 hours, the volume of each solution consumed was recorded. The percentage of sucrose consumption = (sucrose consumption in g/total fluid consumption in g) × 100%.
Rats were deeply anesthetized with sodium pentobarbital (40 mg/kg, intraperitoneal injection) and decapitated. The hippocampus was removed on ice, weighed, and homogenized in methyl alcohol/water (v/v = 50 : 50), then centrifuged at 3000 rpm for 30 minutes at -4 ℃, and the resulting supernatant was analyzed. Quantification of glutamate was performed by high-performance liquid chromatography (Agilent Technologies, USA) with fluorescence detection on a Hypersil ODS column (Dalian Elite Analytical Instruments, China) ( C18, 4.6 mm × 250 mm, 5 μm). The mobile phase included Mobile Phase A (0.05 mmol/L sodium acetate buffer, pH 6) and Mobile Phase B (acetonitrile/water, V/V = 50 : 50). The column temperature was kept at 28 °C, and pump flow rate was maintained at 1.0 mL/minute.
The hippocampus was homogenized in ice-cold radioimmunoprecipitation assay buffer. The bicinchoninic acid method was used to detect protein concentration. An equal amount of protein (30 μg) was loaded for each lane, separated by sodium dodecyl sulfate-polyacrylamide gel electrophoresis (10% gel), and transferred onto polyvinylidene fluoride membranes (Millipore, Billerica, MA, USA). Next, membranes were blocked with 5% skimmed milk at room temperature for two hours and incubated overnight at 4 °C with primary antibodies at a dilution of mouse 1 : 2000 anti-Bax (Affbiotech, Changzhou, China), mouse 1 : 1000 anti-Bcl-2 (Affbiotech, Changzhou, China), mouse 1 : 1000 anti-Caspase 3 (Abcam, Cambridge, UK) and mouse 1 : 2000 anti-CaMKⅡ (Affbiotech, Changzhou, China). After washing with phosphate-buffered solution, membranes were incubated with secondary antibody horseradish peroxidase-conjugated goat anti-mouse immunoglobulin G 1 : 2000 (SA00001-15, Proteintech, USA) for one hour, followed by extensive washing. Proteins were analyzed with an enhanced chemiluminescence system (ECL kit, Pierce Biotechnology, Beijing, China) and recorded by light-sensitive X-ray film (AGFA, Belgium). Optical densities were detected by Image J software (National Institutes of Health, Bethesda, MD, USA). Glyceraldehyde-3-phosphate dehydrogenase (GAPDH) provided the loading control.
Brain tissue was cut into 35 μm slices by freezing microtome (Microm, Thermo Fisher Scientific, Waltham, MA, USA) until the entire structure of the hippocampus was detected. Slices were washed three times with 0.1 M phosphate-buffered saline (PBS), and incubated at room temperature for 20 minutes in 5% bovine serum albumin. Primary (overnight, 4 °C) and secondary antibody incubation (one hour, room temperature) were carried out. Slices were washed three times with PBS after each incubation. Primary antibodies included mouse Anti-NeuN 1 : 500 (ab177487, Abcam), mouse Anti-GFAP 1 : 500 (16825-1-AP, Proteintech), mouse Anti-NMDAR2A 1 : 200 (ab174636, Abcam) and mouse Anti-NMDAR2B 1 : 200 (ab93610, Abcam). Secondary antibodies included goat anti-mouse immunoglobulin G (Alexa Fluor 647) 1 : 2000 (ab150167, Abcam), and goat anti-mouse immunoglobulin G (Alexa Fluor 488) 1 : 2000 (ab150157, Abcam). Slices were coverslipped with a water-based mounting medium containing 4',6-diamidino-2-phenylindole (DAPI) (KeyGEN BioTECH, Jiangsu, China). Stained sections were then observed by fluorescence microscopy (Olympus) processed with Image J Software.
Statistical Product and Service Solutions (SPSS) 24.0 was adopted for data analysis. Results are given as mean±standard error of the mean (SEM). Data normality was assessed by the Kolmogorov-Smirnov test. Differences in HPLC, Western blot and immunofluorescence analysis between the groups were compared by one-way analysis of variance (ANOVA) with the least significant difference (LSD). For the homogeneity analysis, the F test was employed to evaluate the equality of variances in t-test and Levene’s test of equality of error variances for ANOVA. For statistical analyses of the behavioral tests within each group, a paired t-test was employed. A P-value < 0.05 was considered statistical significance.
The bodyweight of the control group increased significantly over time, but in contrast, the other three groups decreased. A prominent decrease was observed on Day 21 (P = 0.003; Fig. 1A) and Day 28 (P = 0.003; Fig. 1A) in the 28-day CUMS group, compared to the weight change in the same group on Day 7.
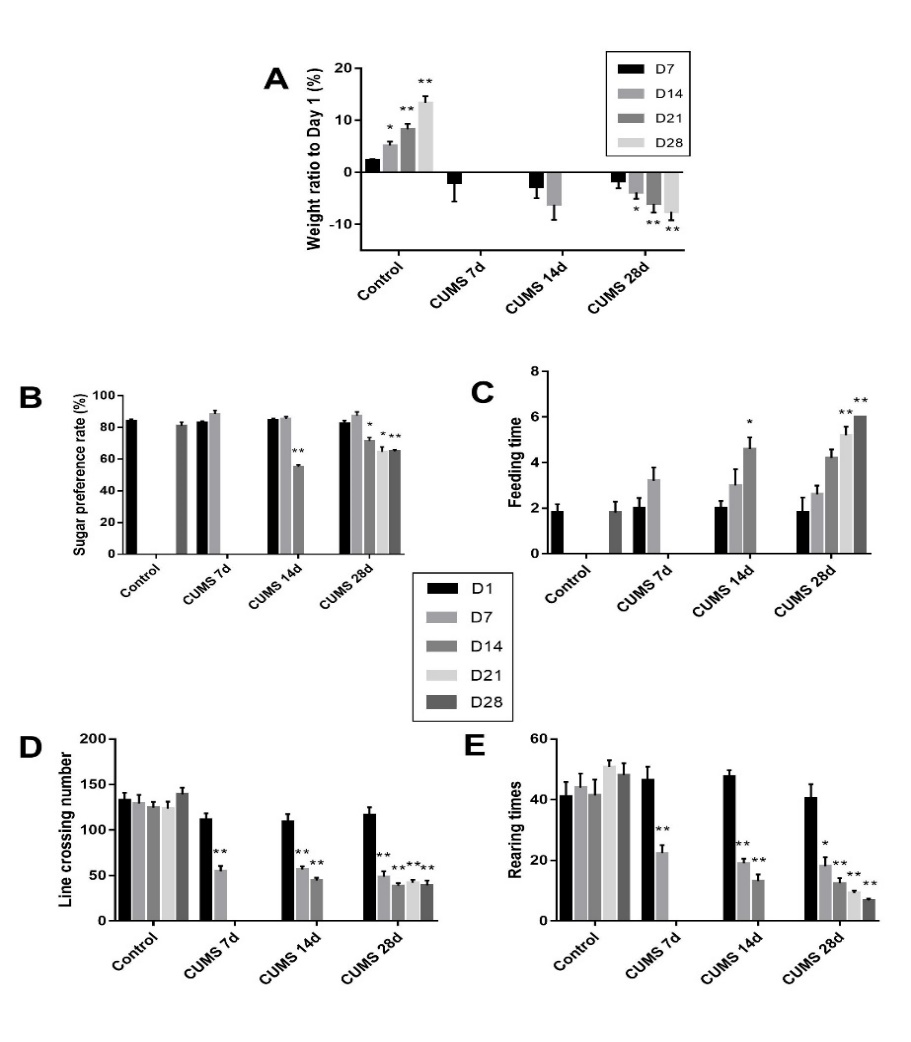
The effect of CUMS on body weight and behavior. (A): Bodyweight change for each group at different time points relative to the first day. *P < 0.05, **P < 0.01 vs. Day 7 in the same group. (B): Sucrose preference rate, (C): The time to first bite, (D): Number of line crossings and E: Times of rearing at specified time points for each group. *P < 0.05, **P < 0.01 vs. Day 1 in the same group. Data are presented as mean±SEM, n = 10 for each group.
Except for the control group, rats were deprived of food and water for 24 hours before the sucrose preference test. Consequently, the control group was only tested on Day 1 and 28. No significant difference in sucrose preference rate was found in the control group. There was no significant difference between Day 1 and 7 in the 7-day CUMS group, 14-day CUMS group, and 28-day CUMS group, but a significant reduction was found on Day 14 for the 14-day CUMS group (P < 0.001; Fig. 1B) and on Day 14 (P = 0.021; Fig. 1B), 21 (P = 0.016; Fig. 1B) and 28 (P = 0.002; Fig. 1B) for the 28-day CUMS group, when compared with the sucrose consumption rate on Day 1 for the same group.
Except for the control group, rats had fasted for 24 hours before the novelty suppressed feeding test. Consequently, the control group was only tested on Day 1 and 28. The time to first bite for the control group was shorter than that of the other three groups. There was no significant difference between Day 1 and 28 for the control group and no significant difference within the 7-day CUMS group. A difference was found on Day 14 for the 14-day CUMS group (P = 0.025; Fig. 1C), compared to the time to first bite on Day 1 for the same group. The time increased significantly on Day 21 (P = 0.007; Fig. 1C) and Day 28 (P = 0.003; Fig. 1C) in the 28-day CUMS group. On Day 28 in the 28-day CUMS group, no rat consumed any food within six minutes, but the time to first bite was recorded as 6 six minutes.
There was no significant difference in the number of line crossing and the times of rearing in the control group. However, there was a significant difference on Day 7 and after in the 7-day CUMS group, 14-day CUMS group, and 28-day CUMS group (P < 0.01; Fig. 1D and 1E).
According to the one-way ANOVA, there were significant differences in glutamate levels between the four groups (F = 30.947, P < 0.001; see Fig. 2). The glutamate concentration increased remarkably in the 7-day CUMS group (25.696 + 2.199 µg/mg, P = 0.004; Fig. 2), 14-day CUMS group (28.851 + 3.483 µg/mg, P < 0.001; Fig. 2) and 28-day CUMS group (37.333 + 3.899 µg/mg, P < 0.001; Fig. 2), compared to control (19.433 + 1.922 µg/mg).
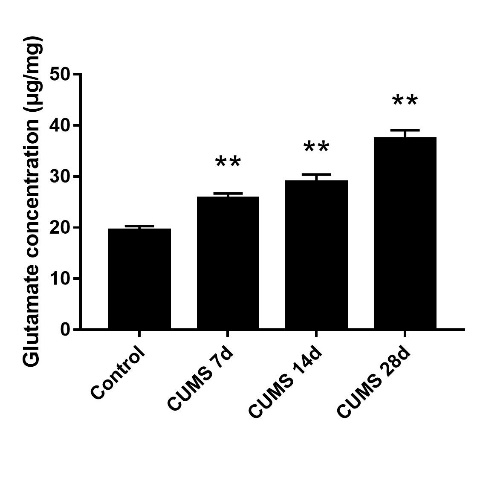
The content of glutamate in each group. Values are presented as mean±SEM; n = 5 for each group; One-way ANOVA. **P < 0.01 compared to control. The glutamate concentration increased remarkably in the other three groups compared to the control group.
The protein expression levels of CaMKⅡ, Bax, and caspase 3 were up-regulated, and the protein content increased with the time of modeling, but Bcl-2, on the contrary. Compared with the control group, the relative ratio of CaMKⅡ rose along with the time of modeling (F[3,16] = 42.113, P < 0.001; Fig. 3), same with Bax (F[3,16] = 31.575, P < 0.001; Fig. 3) and caspase 3 (F[3,16] = 44.105, P < 0.001; Fig. 3), but Bcl-2 was down-regulated (F[3,16] = 61.155, P < 0.001; Fig. 3).
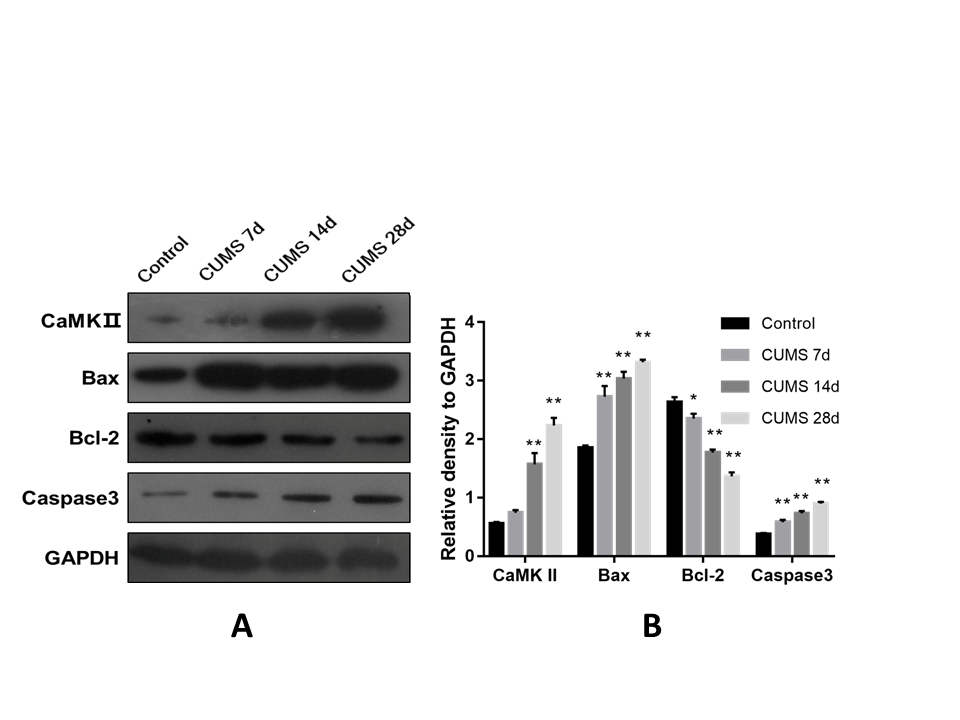
Effect of CUMS on the expression of CaMKⅡ, Bax, Bcl-2, and caspase 3 in hippocampus. (A): Western blot analyses of CaMKⅡ, Bax, Bcl-2, and caspase 3 protein levels in hippocampal homogenates. (B): Protein expression ratio relative to GAPDH. Data given as mean±SEM, n = 5 for each group. *P < 0.05 and **P < 0.01 compared to control.
In comparison to control, the expression of NR2A (F[3,16] = 18.160, P < 0.001; Fig. 4A and C) in neurons decreased significantly and that of NR2B (F[3,16] = 23.346, P < 0.001; Fig. 4B and D) in astrocytes increased significantly with the prolongation of modeling time in the model groups. A gradual reduction of NeuN (F[3,16] = 49.913, P < 0.001; Fig. 4A and C) and GFAP (F[3,16] = 95.114, P < 0.001; Fig. 4B and D) were observed with the time of modeling.
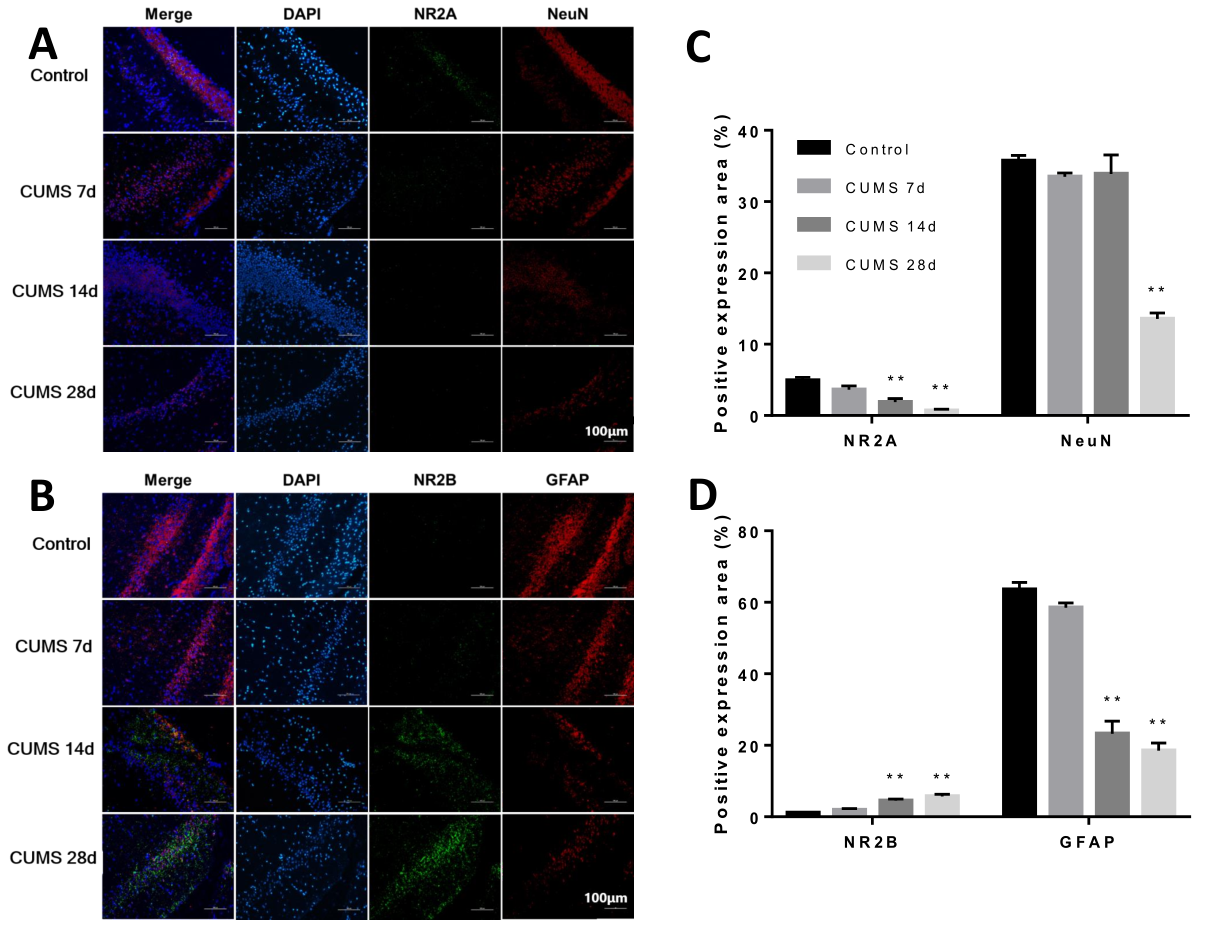
Double-immunofluorescence micrographs show (A) NR2A and NeuN positive cells and (C) NR2B and GFAP positive cells in the hippocampus for each group. Positive expression area (%) of (B) NR2A and NeuN and (D) NR2B and GFAP. Mean±SEM, n = 5 for each group. *P < 0.05 and **P < 0.01 compared to control.
In the current study, a CUMS rat model was employed to investigate the interrelationships between the glutamate system, glia, and depression, further, to probe into the underlying mechanism of astrocyte impairment in depression. Chronic stress might induce excessive glutamate release within the synapse, thus contribute to overstimulation of NMDA receptors, followed by the elevation of intracellular astrocytic calcium levels leading to subsequent mediation of the astrocyte apoptotic pathway. Calcium overload in astrocytes may be the underlying mechanism of astrocyte impairment in depression. Accordingly, further research with large samples and novel approaches is required to elucidate any specific interaction occurring between the intracellular calcium level and astrocyte impairment in depression.
The content of glutamate in the 7-day CUMS group, 14-day CUMS group, and 28-day CUMS group was shown to increase significantly with time compared to a control group (P < 0.01). This suggested that depression in rats may be relevant to changes in glutamate levels in the hippocampus.
A prominent reduction in body weight was observed after 21 days, and a remarkable drop in sucrose preference and protracted latency of eating in novelty suppressed feeding test was found after 14 days of CUMS in the model groups. There was also a notable decrease in horizontal and vertical movement in OFT after 7 days of CUMS in the model groups. All these results indicated to confirm that a 21-day-long CUMS procedure was sufficient to induce a depressive animal model.
Up-regulated levels of Bax and caspase 3 and down-regulated levels of Bcl-2 were demonstrated within the duration of the model, which indicated apoptosis in the hippocampus. The result of NeuN and GFAP staining showed significant neuronal and astrocytic loss to subsequently exist in the hippocampus. Although recent work suggests chronic stress may result in depression by impairing astrocytes, the underlying mechanisms remain unclear.
The relative ratio of CaMKⅡ increased with model duration. This was taken to indicate a rise of intracellular calcium concentration, which itself has been taken to implicate the initiation and occurrence of cell death (Orrenius et al., 2015) and in turn, could trigger the pivotal steps in the apoptotic process (Orrenius et al., 2003). Accordingly, there was a close association between intracellular Ca2+ overload and cell death (Orrenius et al., 2015).
CUMS, chronic unpredictable mild stress; OFT, open field test; CaMKII, calcium/calmodulin-dependent protein kinase II; Bcl-2, B-cell lymphoma-2; NMDA, N-methyl-D-aspartic acid; NR2A, glutamate ionotropic receptor NMDA type subunit 2A; NR2B, glutamate ionotropic receptor NMDA type subunit 2B; NeuN, neuronal nuclear protein; GFAP, glial fibrillary acidic protein; HPLC, high performance liquid chromatography; GAPDH, glyceraldehyde-3-phosphate dehydrogenase; PBS, phosphate-buffered saline; DAPI, 4',6-diamidino-2-phenylindole; SEM, standard error of mean; ANOVA, analysis of variance; LSD, least significant difference.
This work was supported by the Zhejiang Natural Science Foundation [grant numbers LY17H270009, LY18H270005]; the National Natural Science Foundation of China [grant number 81503646].
The authors declare no competing interests.