†These authors contributed equally.
Academic Editor: Gianluca Campo
Background: Remote ischemic preconditioning (RIPC) has cardioprotective effects. This study was designed to evaluate the effectiveness and potential influencing factors of RIPC for myocardial ischemia-reperfusion injury (MIRI) in rats and mice. Methods: The PubMed, Web of Science, Embase, and Cochrane Library databases were searched to identify animal model studies that explored the effect of RIPC on MIRI. The primary outcome was myocardial infarct size, and secondary outcomes included serum cardiac markers, vital signs, hemodynamic parameters, and TUNEL-positive cells. Quality was assessed using SYRCLE’s Risk of Bias Tool. Results: This systematic review and meta-analysis included 713 male animals from 37 studies. RIPC significantly protected against MIRI in small animal models by reducing infarct size, decreasing serum myocardial marker levels and cell death, and improving cardiac function. Subgroup analysis indicated that RIPC duration and sites influence the protective effect of RIPC on MIRI. Meta-regression suggested that study type and staining method might be sources of heterogeneity. The funnel plot, Egger’s test, and Begg’s test suggested the existence of publication bias, but results of the sensitivity analysis and nonparametric trim-and-fill method showed that the overall effect of RIPC on MIRI infarct size was robust. Conclusions: RIPC significantly protected against MIRI in small animal models by reducing infarct size, decreasing serum myocardial markers and limiting cell death, and improving cardiac function. RIPC duration and site influence the protective effect of RIPC on MIRI, which contributes in reducing confounding factors and determines the best approach for human studies.
Cardiovascular diseases are major contributors to the disease burden, with acute myocardial infarction being the most severe manifestation. Disability-adjusted life years due to ischemic heart disease have reached 182 million, which increased steadily from 1990 to 2019, causing global mortality and rise in healthcare costs [1]. Reperfusion and revascularization strategies, such as percutaneous coronary intervention and coronary artery bypass grafting, have been widely used to improve perfusion and prevent acute re-occlusion in acute myocardial infarction [2]. However, reperfusion could also lead to additional injury, including increased infarct size and microvascular dysfunction [3]. This is called myocardial ischemia-reperfusion injury (MIRI). MIRI is generally related to calcium overload, increased reactive oxygen species, proinflammatory factors, endoplasmic reticulum stress, and mitochondrial dysfunction [4], leading to different forms of cell death [5].
Murry first reported ischemic preconditioning in 1986 as a non-pharmacological intervention for MIRI [6]. Remote ischemic preconditioning (RIPC), brief and transient episodes of ischemia at a remote site before myocardial ischemia, have been reported to have a cardioprotective effect [7]. Some clinical experiments have explored the cardioprotective effect of RIPC in patients undergoing surgery; the results were controversial, which might be attributed to confounding factors, such as age, comorbidities, surgery, anesthesia, medication, and RIPC method [8, 9, 10, 11, 12]. Therefore, finding an ideal protocol, including the appropriate site, duration, and cycles of RIPC, and exploring the factors influencing its protective effect from preclinical evidence are important for optimizing RIPC in clinical studies. Although multiple animal studies have been conducted to explore the effect and mechanism of RIPC on MIRI, there is still a lack of systematic reviews and meta-analyses to assess the overall effect of RIPC on MIRI in small animal studies. Therefore, this study was designed to evaluate the effects and potential influencing factors of RIPC on MIRI in rats and mice.
This systematic review and meta-analysis was performed according to the PRISMA guidelines and registered in PROSPERO (CRD42022362017). Four databases, including PubMed, Web of Science, Embase, and Cochrane Library, were searched until June 13, 2022, to identify animal studies exploring the effect of RIPC on MIRI. The search keywords were “Remote ischemic preconditioning”, “RIPC”, “myocardial ischemia-reperfusion injury”, “MIRI”, “MIR”, and “myocardial reperfusion injury”.
The inclusion criteria were as follows: (1) studies on young male rats or mice; (2) studies using in vivo or ex vivo models of MIRI; (3) where animals in the treatment group received RIPC, while the control group received a placebo or no treatment; (4) myocardial infarction size was measured by triphenyl tetrazolium chloride (TTC) staining and reported as a percentage; and (5) language limited to English. The exclusion criteria were as follows: (1) studies on animals with other comorbidities such as diabetes or hyperlipidemia; (2) having incomplete data; (3) duplicate publications; (4) review, conference abstract, comment, and protocol; and (5) studies where animals received substances obtained from the blood of humans who received RIPC.
After removing duplicates, two authors screened for eligibility by browsing the titles and abstracts of the records, followed by the full text. Another author was consulted in case of any disagreement. After confirming the included studies, two authors extracted the data independently using Excel 2019 (Microsoft Corp., Redmond, WA, USA), and disagreements were resolved by another author. Study characteristics were extracted, including author names, year of publication, country, species, animal weight, number of animals in both groups, anesthesia method, ischemia/reperfusion (I/R) method, I/R duration, RIPC protocol, RIPC site, outcome measurement, and staining method. The primary outcome was myocardial infarct size, and secondary outcomes included serum cardiac markers, vital signs, hemodynamic parameters, and TUNEL-positive cells. If there were any missing data or the data were presented in figures, the corresponding author would be contacted for more information. Data, including the number, mean, and standard deviation (SD), were collected. If there was only a standard error of the mean (SEM) reported in the articles, SEM was transformed into SD.
Two authors evaluated the quality of the included studies using SYRCLE’s Risk of Bias Tool [13] which is recommended for animal studies. A third author was invited to resolve any disagreements. This tool assessed six domains of bias: selection bias (sequence generation, baseline characteristics, and allocation concealment), performance bias (random housing and blinding), detection bias (random outcome assessment and blinding), attrition bias (incomplete outcome data), reporting bias (selective outcome reporting), and other sources of bias. Each entry was evaluated as unclear, low risk, or high risk. The higher the score, the better the quality of the study.
Stata version 12.0 (Stata Corp., College Station, TX, USA) was used for the
meta-analysis. The standardized mean difference with a 95% confidence interval
(95% CI) was used to evaluate the difference between the RIPC and control
groups. Heterogeneity was evaluated using Cochran’s Q test and Higgins’ I
A total of 594 studies were identified from PubMed, Web of Science, Embase, and Cochrane Library databases. After removing duplicates, 440 studies underwent title and abstract screening, and 326 were excluded. The full texts of the remaining 114 studies were assessed. Seventy-seven studies were excluded; 38 owing to incomplete data, 24 owing to unrelated data, 1 was an in vitro study, 1 reported on other comorbidities, 9 were in another language, and 4 were on female animals. Finally, 37 articles were included in the quantitative synthesis [14, 15, 16, 17, 18, 19, 20, 21, 22, 23, 24, 25, 26, 27, 28, 29, 30, 31, 32, 33, 34, 35, 36, 37, 38, 39, 40, 41, 42, 43, 44, 45, 46, 47, 48, 49, 50] (Fig. 1).
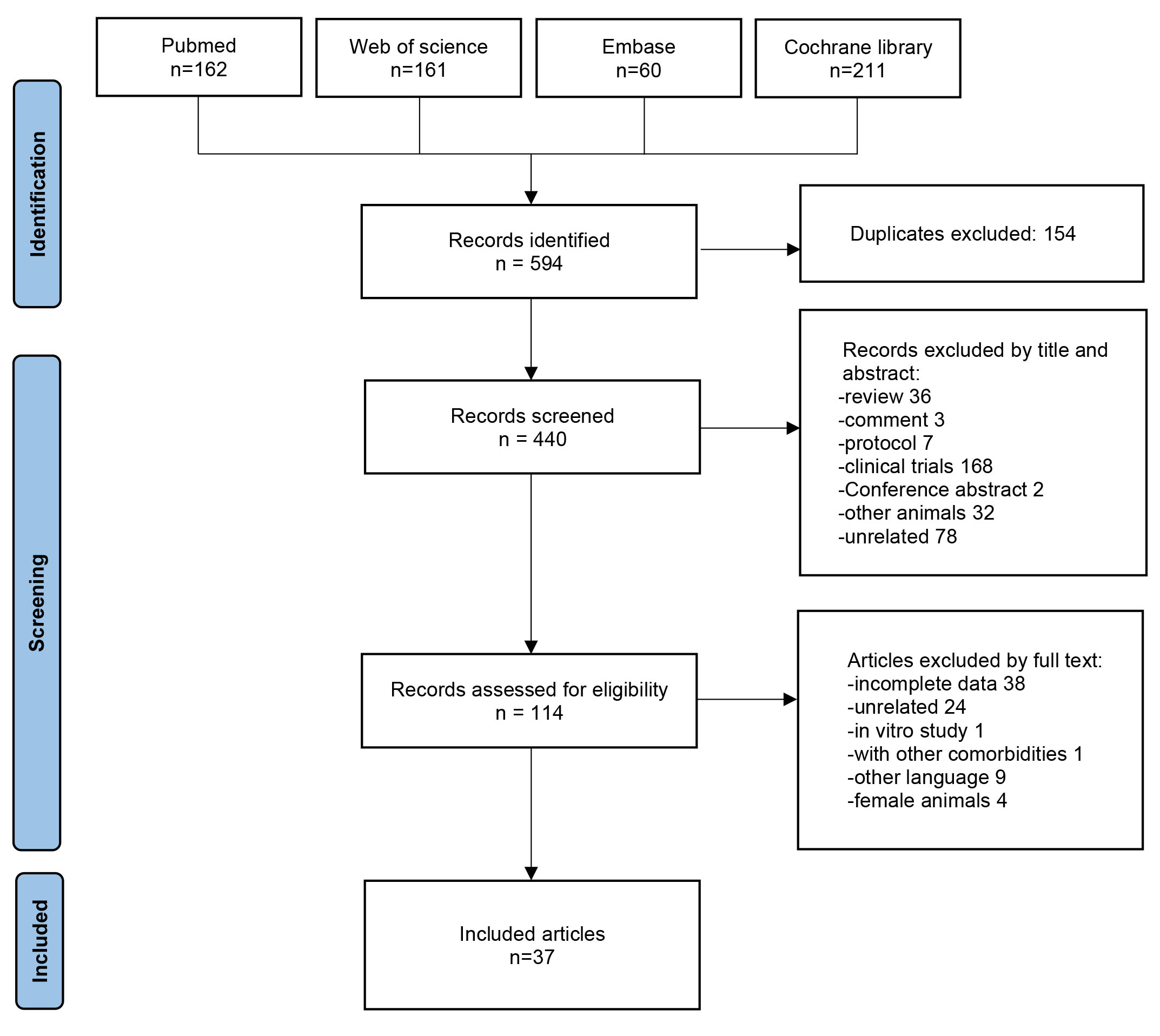
Prisma chart flow. A total of 594 studies were identified from Pubmed, Web of Science, Embase, and Cochrane library. After assessed of eligibility, 37 articles were included in the quantitative synthesis.
The meta-analysis included 37 published articles from which we extracted data of 45 comparative studies between RIPC and control groups in MIRI models, and the characteristics of the articles are shown in Table 1 (Ref. [14, 15, 16, 17, 18, 19, 20, 21, 22, 23, 24, 25, 26, 27, 28, 29, 30, 31, 32, 33, 34, 35, 36, 37, 38, 39, 40, 41, 42, 43, 44, 45, 46, 47, 48, 49, 50]). These articles were published in China, Germany, France, Australia, India, Argentina, America, Japan, Slovakia, Denmark, Spain, the Netherlands, the Russian Federation, and the United Kingdom from 2005 to 2021. A total of 27 articles used the in vivo MIRI model by ligation and release of the left anterior descending coronary artery, and the remaining 10 used the ex vivo MIRI model by Langendorff perfusion. A total of 713 animals were included in this meta-analysis, including Friend Virus Bmice, C57Bl/6 mice, Wistar rats, Sprague–Dawley rats, and Lewis rats. The RIPC site included the limb, femoral artery, infrarenal aorta, mesenteric artery, hepatic vessels, and abdominal aorta.
Author | Year | Country | Species | Weight | RIPC/control | Anesthesia | I/R method | I/R duration | RIPC site | RIPC protocol | Outcome measurements | Staining method |
---|---|---|---|---|---|---|---|---|---|---|---|---|
Ren [14] | 2021 | China | FVB mice | unclear | 6/6 | 50 mg/kg thiopental | ex vivo | 30/90 min | hepatic occlusion of the portal triad | 5/5 min 3 cycles | infarct size, CK-MB, LDH, HR, LVDP | TTC |
Lucia [15] | 2021 | Slovakia | Wistar rats | 250 |
8/8 | 50–60 mg/kg thiopental | ex vivo | 30/120 min | right hind limb by pressure cuff (200 mmHg) | 5/5 min 3 cycles | infarct size, recovery of LVDP, duration of VT | TTC |
Marie [16] | 2021 | Denmark | SD rats | 300 g | 8/7 | 65 mg/kg pentobarbital | ex vivo | 30/120 min | right hind limb (tourniquet) | 5/5 min 3 cycles | infarct size, HR, LVDP, RPP, CF, LDH | TTC |
Yasuaki [17] | 2020 | Japan | Wistar rats | unclear | 8/8 | 2 mg/kg midazolam, 2.5 mg/kg butorphanol and 0.15 mg/kg medetomidine | in vivo | 30/120 min | right forelimb and hindlimb | 5/5 min 3 cycles | infarct size, HR, MAP | TTC-Evans blue |
Ke [18] | 2020 | China | SD rats | 250–300 g | 6/6 | 50 mg/kg pentobarbital | in vivo | 30/120 min | unilateral hindlimb | 5/5 min 3 cycles | infarct size, HR, MAP, RPP | TTC-Patent blue |
Billah [19] | 2020 | Australia | SD rats | 300–350 g | 8/8 | 2–5% isoflurane | in vivo | 30 min/24 h | hindlimb | 5/5 min 3 cycles | infarct size | TTC-Evans blue |
Diamela [20] | 2019 | Argentina | SD rats | 200–250 g | 6/6 | 65 mg/kg urethane | ex vivo | 30/120 min | femoral artery occlusion | 5/5 min 3 cycles | infarct size | TTC |
Billah [21] | 2019 | Australia | SD rats | 300–350 g | 8/10 | 2–5% isoflurane | in vivo | 30 min/24 h | hindlimb | 5/5 min 3 cycles | infarct size | TTC-Evans blue |
Sapna [22] | 2019 | India | Wistar rats | 150–220 g | 6/6 | 50 mg/kg thiopental | ex vivo | 30/120 min | hindlimb | 5/5 min 4 cycles | infarct size, LDH, CK, LVDP, dp/dt max, dp/dt min | TTC |
Patrick [23] | 2018 | Germany | Wistar rats | 293 |
9/10 | 100 mg/kg pentobarbital | in vivo | 25/120 min | bilateral hind-limb ischemia by blood pressure cuff (200 mmHg) | 5/5 min 4 cycles | infarct size, HR, MAP | TTC-Evans blue |
Xavier [24] | 2018 | Spain | C57Bl/6 mice | unclear | 16/19 | 60 mg/kg pentobarbital | in vivo | 40/120 min | right hindlimb vascular occlusion | 5/5 min 3 cycles | infarct size | TTC-Evans blue |
Helmut [25] | 2018 | Germany | Lewis rats | 200–380 g | 16/8 | 100 mg per 10 mg/kg ketamine/xylazine | ex vivo | 30/120 min | left hindlimb | 5/5 min 3 cycles | infarct size | TTC-Patent blue |
Chen [26] | 2018 | China | Mice | unclear | 8/7 | 2–5% isoflurane | in vivo | 30/180 min | left femoral artery occlusion | 5/5 min 3 cycles | infarct size, TUNEL-positive cells, CK, CK-MB, LDH | TTC-Evans blue |
Friederike [27] | 2018 | Netherlands | Wistar rats | 301 |
6/6 | 40 mg/kg/h pentobarbital | in vivo | 25/120 min | bilateral hind-limb | 5/5 min 4 cycles | infarct size, HR, MAP | TTC-Evans blue |
6/6 | Sevoflurane (1 minimal alveolar concentration) + remifentanil (0.5 µg/kg/min) | |||||||||||
6/6 | Propofol (12 mg/kg/h) + remifentanil (0.5 µg/kg/min) | |||||||||||
Yu [28] | 2017 | China | SD rats | 250–300 g | 10/10 | ethyl carbamate | ex vivo | 30/60 min | unilateral hindlimb | 5/5 min 4 cycles | infarct size, HR, LVEDP, RPP, dp/dt max, dp/dt min, cTNI | TTC |
Yang [29] | 2017 | China | SD rats | 250–300 g | 5/5 | 50 mg/kg pentobarbital | in vivo | 45/180 min | hepatic vessel clamp | 5/5 min 3 cycles | infarct size, serum LDH and CK-MB, LVSP, LVEDP, dp/dt max, dp/dt min, TUNEL-positive cells | TTC |
Amritpal [30] | 2017 | India | Wistar rats | 150–220 g | 6/6 | 50 mg/kg thiopental | ex vivo | 30/120 min | hindlimb by blood pressure cuff (150 mmHg) | 5/5 min 4 cycles | infarct size, LDH, CK, LVDP, dp/dt max, dp/dt min | TTC |
Mudaliar [31] | 2017 | Australia | SD rats | 250–300 g | 7/7 | unclear | in vivo | 30 min/24 h | hindlimb | 5/5 min 3 cycles | infarct size | TTC-Evans blue |
Friederike [32] | 2017 | Germany | Wistar rats | 263 |
6/6 | 100 mg/kg pentobarbital | in vivo | 25/120 min | bilateral hind-limb ischemia by blood pressure cuff (200 mmHg) | 5/5 min 4 cycles | infarct size, HR, MAP | TTC |
Michael [33] | 2016 | Russian Federation | Wistar rats | 220–260 g | 7/7 | 60 mg/kg pentobarbital | in vivo | 30/90 min | infrarenal aorta occlusion | 5/15 min 1 cycle | infarct size, VT/VF duration, mortality rates, HR, MAP | TTC-Evans blue |
7/7 | 15/15 min 1 cycle | |||||||||||
6/7 | 30/15 min 1 cycle | |||||||||||
12/14 | mesenteric artery occlusion | 15/15 min 1 cycle | ||||||||||
6/10 | 15/15 min 1 cycle | |||||||||||
Donato [34] | 2016 | Argentina | Wistar rats | 200–250 g | 10/8 | 65 mg/kg pentobarbital | ex vivo | 30/120 min | left femoral artery occlusion | 5/5 min 3 cycles | infarct size | TTC |
Juan [35] | 2016 | France | Wistar rats | unclear | 10/8 | 60 mg/kg pentobarbital | in vivo | 40/120 min | upper right femoral artery occlusion | 5/5 min 4 cycles | infarct size | TTC |
Laura [36] | 2016 | France | Wistar rats | 200–250 g | 6/6 | 60 mg/kg pentobarbital | in vivo | 40/120 min | upper right femoral artery occlusion | 5/5 min 4 cycles | infarct size | TTC-Evans blue |
Chai [37] | 2015 | China | SD rats | 250–300 g | 14/14 | 50 mg/kg pentobarbital | in vivo | 30/180 min | bilateral femoral artery occlusion | 5/5 min 3 cycles | infarct size, HR, MAP, Serum cTNI, TUNEL-positive cells | TTC-Evans blue |
Tienush [38] | 2014 | Germany | C57Bl/6 mice | 32 |
5/5 | 45 mg/kg ketamine and 10 mg/kg xylazine | in vivo | 30 min/24 h | right upper hindlimb | 5/5 min 4 cycles | infarct size | TTC-Evans blue |
Hussein [39] | 2014 | France | Wistar rats | 200–250 g | 6/7 | 60 mg/kg pentobarbital | in vivo | 40/120 min | upper right femoral artery occlusion | 5/5 min 4 cycles | infarct size | TTC-Evans blue |
Chai [40] | 2014 | China | SD rats | 250–300 g | 15/15 | 50 mg/kg pentobarbital | in vivo | 30/180 min | bilateral femoral arteries occlusion | 5/5 min 3 cycles | infarct size, HR, MAP, serum CK-MB, cTNI, LDH, TUNEL-positive cells | TTC-Evans blue |
15/15 | abdominal aorta occlusion | |||||||||||
Timo [41] | 2014 (1) | Germany | Wistar rats | unclear | 6/6 | 100 mg/kg pentobarbital | in vivo | 35/120 min | bilateral hind limb | 5/5 min 4 cycles | infarct size, HR, MAP | TTC-Evans blue |
Timo [42] | 2014 (2) | Germany | Wistar rats | unclear | 6/6 | 80 mg/kg pentobarbital | in vivo | 35/120 min | bilateral hind limb | 5/5 min 4 cycles | infarct size, HR, MAP | TTC-Evans blue |
Zhu [43] | 2013 | China | Wistar rats | 340 |
6/6 | 3 mL/kg 10% chloral hydrate | in vivo | 30/180 min | bilateral hind limb | 5/5 min 3 cycles | infarct size, arrhythmic score, LVSP, LVEDP, dp/dt max, dp/dt min | TTC-Evans blue |
Pierre [44] | 2013 | France | Wistar rats | unclear | 11/9 | 60 mg/kg pentobarbital | in vivo | 40/120 min | upper right femoral artery occlusion | 10/10 min 1 cycle | infarct size | TTC-Evans blue |
Cai [45] | 2013 | America | Mice | unclear | 6/6 | 70 mg/kg pentobarbital | in vivo | 30/120 min | left femoral artery occlusion | 5/5 min 3 cycles | infarct size | TTC-Evans blue |
Duan [47] | 2012 | China | SD rats | 300–350 g | 5/5 | 50 mg/kg pentobarbital | ex vivo | 30/60 min | bilateral femoral artery occlusion | 5/5 min 3 cycles | infarct size, TUNEL-positive cells, HR, LVDP, CF, dp/dt max, dp/dt min | TTC |
Lu [46] | 2012 | China | SD rats | 280–300 g | 6/6 | 50 mg/kg pentobarbitone | in vivo | 30/120 min | right femoral artery by vessel clip | 5/5 min 1 cycle | infarct size, HR, MAP, RPP, CF | TTC-Evans blue |
6/6 | 5/5 min 3 cycles | |||||||||||
Nicole [48] | 2011 | Germany | Wistar rats | 300–350 g | 6/6 | 80 mg/kg pentobarbital | in vivo | 35/120 min | hindlimb | 5/5 min 4 cycles | infarct size, HR, MAP, serum CK and TnT | TTC-Evans blue |
Shiang [49] | 2010 | United Kingdom | C57Bl/6 mice | 25–30 g | 9/10 | 0.01 mL/g of 10 mg/mL ketamine, 2 mg/mL xylazine and 0.06 mg/mL atropine | in vivo | 30/120 min | left femoral artery occlusion | 5/5 min 3 cycles | infarct size, HR, MAP | TTC-Evans blue |
Sebastian [50] | 2005 | Germany | Wistar rats | 290–350 g | 6/6 | 70 mg/kg pentobarbital | in vivo | 30/150 min | mesentery artery occlusion | 15/15 min 1 cycle | infarct size, MAP | TTC-Black Chinese ink |
Abbreviation: RIPC, remote Ischemic preconditioning; I/R, ischemia/reperfusion; TTC, 2,3,5-Triphenyltetrazolium chloride; CK, creatine kinase; LDH, lactate dehydrogenase; cTnI, cardiac troponin I; TnT, troponin T; HR, heart rate; MAP, mean arterial pressure; RPP, heart rate-blood pressure product; LVDP, left ventricular developed pressure; LVSP, left ventricular systolic pressure; LVEDP, left ventricular end diastolic pressure; CF, coronary flow; VT, ventricular tachycardia; VF, ventricular fibrillation. |
The SYRCLE Risk of Bias Tool was used to assess the quality of the included studies (Table 2, Ref. [14, 15, 16, 17, 18, 19, 20, 21, 22, 23, 24, 25, 26, 27, 28, 29, 30, 31, 32, 33, 34, 35, 36, 37, 38, 39, 40, 41, 42, 43, 44, 45, 46, 47, 48, 49, 50]). Four studies [33, 37, 40, 43] scored six points, while 10 studies [19, 20, 21, 31, 34, 35, 36, 44, 45, 50] scored only two points. None of the studies described whether allocation concealment was performed, and performance bias (blinding) was at high risk.
Study | A | B | C | D | E | F | G | H | I | J | Total |
Ren 2021 [14] | ? | Y | ? | Y | N | ? | ? | ? | Y | Y | 4 |
Lucia 2021 [15] | ? | Y | ? | Y | N | ? | ? | ? | Y | Y | 4 |
Marie 2021 [16] | ? | Y | ? | Y | N | ? | Y | ? | Y | Y | 5 |
Yasuaki 2020 [17] | ? | Y | ? | Y | N | ? | Y | ? | Y | Y | 5 |
Ke 2020 [18] | ? | Y | ? | ? | N | ? | ? | Y | Y | Y | 4 |
Billah 2020 [19] | ? | ? | ? | ? | N | ? | ? | ? | Y | Y | 2 |
Diamela 2019 [20] | ? | ? | ? | ? | N | ? | ? | ? | Y | Y | 2 |
Billah 2019 [21] | ? | ? | ? | ? | N | ? | ? | ? | Y | Y | 2 |
Sapna 2019 [22] | ? | Y | ? | Y | N | ? | ? | ? | Y | Y | 4 |
Patrick 2018 [23] | ? | Y | ? | ? | N | ? | Y | ? | Y | Y | 4 |
Xavier 2018 [24] | ? | ? | ? | ? | N | ? | ? | Y | Y | Y | 3 |
Helmut 2018 [25] | ? | Y | ? | ? | N | ? | Y | Y | Y | Y | 5 |
Chen 2018 [26] | ? | Y | ? | ? | N | ? | Y | ? | Y | Y | 4 |
Friederike 2018 [27] | ? | Y | ? | ? | N | ? | Y | ? | Y | Y | 4 |
Yu 2017 [28] | ? | Y | ? | Y | N | ? | Y | ? | Y | Y | 5 |
Yang 2017 [29] | ? | Y | ? | Y | N | ? | ? | Y | Y | Y | 5 |
Amritpal 2017 [30] | ? | Y | ? | Y | N | ? | ? | ? | Y | Y | 4 |
Mudaliar 2017 [31] | ? | ? | ? | ? | N | ? | ? | ? | Y | Y | 2 |
Friederike 2017 [32] | ? | Y | ? | ? | N | ? | ? | ? | Y | Y | 3 |
Michael 2016 [33] | ? | Y | ? | Y | N | ? | Y | Y | Y | Y | 6 |
Donato 2016 [34] | ? | ? | ? | ? | N | ? | ? | ? | Y | Y | 2 |
Juan 2016 [35] | ? | ? | ? | ? | N | ? | ? | ? | Y | Y | 2 |
Laura 2016 [36] | ? | ? | ? | ? | N | ? | ? | ? | Y | Y | 2 |
Chai 2015 [37] | Y | Y | ? | Y | N | ? | Y | ? | Y | Y | 6 |
Tienush 2014 [38] | ? | ? | ? | Y | N | ? | ? | ? | Y | Y | 3 |
Hussein 2014 [39] | ? | ? | ? | ? | N | ? | Y | ? | Y | Y | 3 |
Chai 2014 [40] | Y | Y | ? | Y | N | ? | Y | ? | Y | Y | 6 |
Timo 2014 (1) [41] | ? | Y | ? | Y | N | ? | ? | ? | Y | Y | 4 |
Timo 2014 (2) [42] | ? | Y | ? | ? | N | ? | ? | ? | Y | Y | 3 |
Zhu 2013 [43] | ? | Y | ? | ? | N | Y | Y | Y | Y | Y | 6 |
Pierre 2013 [44] | ? | ? | ? | ? | N | ? | ? | ? | Y | Y | 2 |
Cai 2013 [45] | ? | ? | ? | ? | N | ? | ? | ? | Y | Y | 2 |
Duan 2012 [47] | ? | Y | ? | ? | N | ? | ? | ? | Y | Y | 3 |
Lu 2012 [46] | ? | Y | ? | ? | N | ? | ? | Y | Y | Y | 4 |
Nicole 2011 [48] | ? | Y | ? | Y | N | ? | ? | ? | Y | Y | 4 |
Shiang 2010 [49] | ? | Y | ? | ? | N | ? | ? | ? | Y | Y | 3 |
Sebastian 2005 [50] | ? | ? | ? | ? | N | ? | ? | ? | Y | Y | 2 |
Note: Selection bias: A, Sequence generation; B, Baseline characteristics; C, Allocation concealment. Performance bias: D, Random housing; E, Blinding. Detection bias: F, Random outcome assessment; G, Blinding. Attrition bias: H, Incomplete outcome data. Reporting bias: I, Selective outcome reporting. Other: J, Other sources of bias. ?, unclear; Y, low risk; N, high risk. |
The infarct size was analyzed using random effect size because of the high
heterogeneity (I
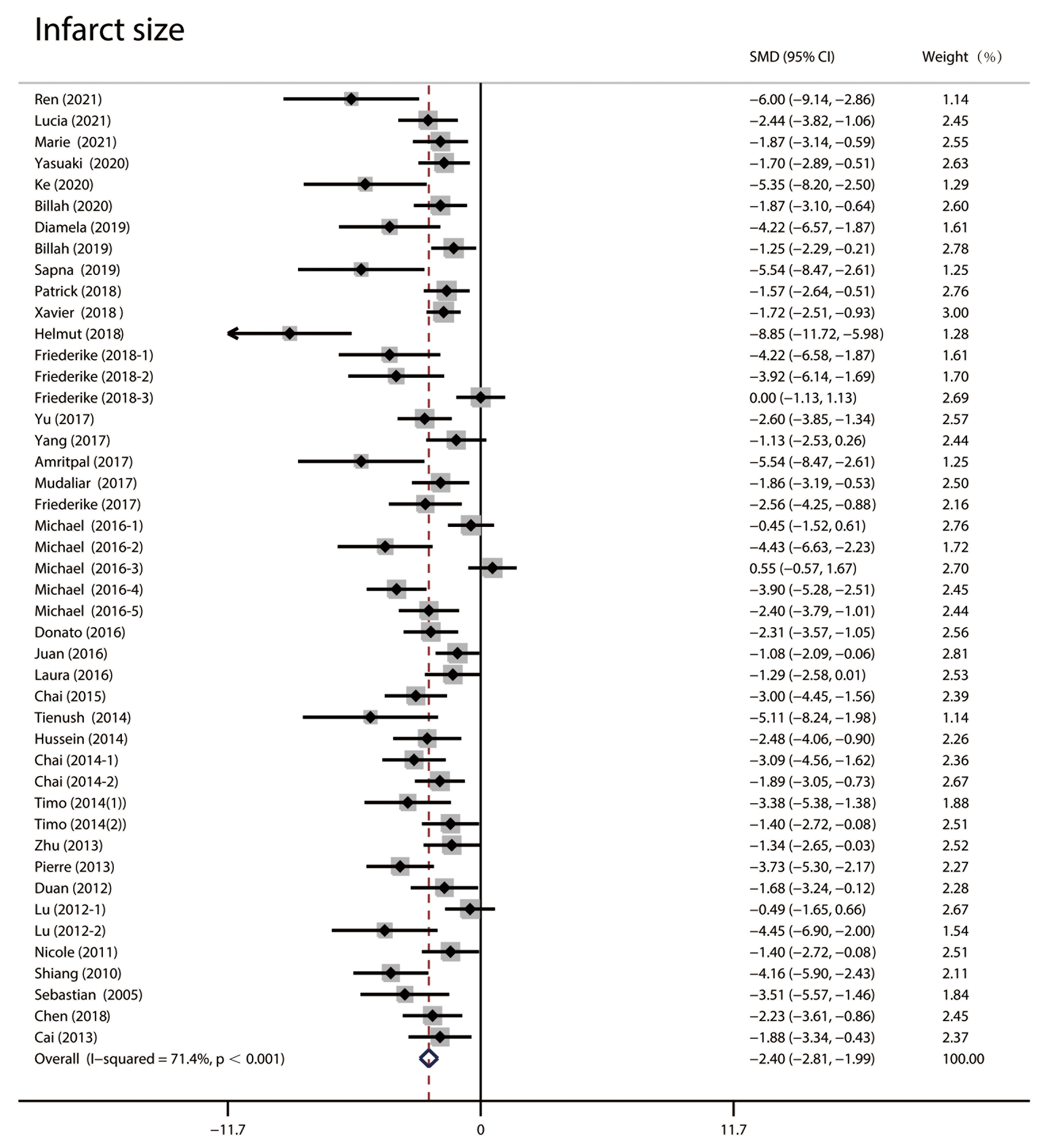
Forest plots of infarct size in random effect size analysis. High heterogeneity was observed, and the infarct size in the RIPC group was significantly lower than in the control group. RIPC, remote Ischemic preconditioning; CI, confidence interval; SMD, standardized mean difference.
Subgroup analysis was performed based on species, study type, anesthesia method,
reperfusion time, staining method, RIPC site, duration, and cycles (Table 3). The
difference between the RIPC and control groups was significant in most subgroups
(p
Subgroup | Number | SMD | 95% CI | Weight % | p value | Heterogeneity | ||
Species | ||||||||
Rats | 39 | –2.328 | –2.775 | –1.881 | 87.77 | 72.20% | ||
Mice | 6 | –2.983 | –4.145 | –1.82 | 12.23 | 67.10% | ||
Anesthesia | ||||||||
Inhalation | 4 | –2.005 | –2.878 | –1.131 | 9.54 | 38.60% | ||
Injection | 40 | –2.473 | –2.935 | –2.011 | 87.96 | 73.80% | ||
Unclear | 1 | –1.86 | –3.186 | –0.534 | 2.5 | 0.006 | NA | |
I/R method | ||||||||
Ex vivo | 10 | –3.638 | –4.765 | –2.512 | 18.94 | 73.60% | ||
In vivo | 35 | –2.118 | –2.539 | –1.697 | 81.06 | 68.10% | ||
Reperfusion duration | ||||||||
1–1.5 h | 8 | –2.368 | –3.701 | –1.036 | 18.05 | 84.90% | ||
2–3 h | 33 | –2.462 | –2.924 | –2.001 | 72.92 | 68.10% | ||
24 h | 4 | –1.911 | –2.863 | –0.958 | 9.02 | 44.10% | ||
RIPC site | ||||||||
Limb | 22 | –2.462 | –3.046 | –1.879 | 47.64 | 70.00% | ||
Hepatic vessel | 2 | –3.354 | –8.103 | 1.395 | 3.58 | 0.166 | 87.00% | |
Femoral artery | 15 | –2.445 | –3.055 | –1.834 | 34.06 | 59.60% | ||
Infrarenal aorta | 3 | –1.216 | –3.411 | 0.978 | 7.18 | 0.277 | 87.20% | |
Mesenteric artery | 2 | –3.151 | –4.621 | –1.681 | 4.88 | 55.30% | ||
Abdominal aorta | 1 | –1.891 | –3.049 | –0.732 | 2.67 | 0.001 | NA | |
RIPC duration | ||||||||
5/5 min | 38 | –2.365 | –2.775 | –1.954 | 83.83 | 65.40% | ||
5/15 min | 1 | –0.451 | –1.517 | 0.615 | 2.76 | 0.407 | NA | |
10/10 min | 1 | –3.734 | –5.297 | –2.171 | 2.27 | NA | ||
15/15 min | 4 | –3.404 | –4.279 | –2.528 | 8.44 | 9.90% | ||
30/15 min | 1 | 0.548 | –0.571 | 1.668 | 2.7 | 0.337 | NA | |
RIPC cycles | ||||||||
1 cycle | 8 | –2.177 | –3.51 | –0.844 | 18.84 | 0.001 | 86.20% | |
3 cycles | 22 | –2.474 | –2.98 | –1.969 | 49.54 | 62.20% | ||
4 cycles | 15 | –2.356 | –3.073 | –1.638 | 31.63 | 67.30% | ||
Staining method | ||||||||
TTC | 12 | –2.556 | –3.287 | –1.825 | 25.06 | 59.00% | ||
TTC-Evans blue | 30 | –2.095 | –2.549 | –1.64 | 70.53 | 69.20% | ||
TTC-patent blue | 2 | –7.098 | –10.528 | –3.668 | 2.57 | 65.30% | ||
TTC-black Chinese ink | 1 | –3.515 | –5.57 | –1.459 | 1.84 | 0.001 | NA | |
Abbreviation: RIPC, remote Ischemic preconditioning; I/R, ischemia/reperfusion; TTC, 2,3,5-Triphenyltetrazolium chloride; SMD, standardized mean difference; CI, confidence interval; NA, not available. |
Meta-regression analysis was performed to detect any possible sources of heterogeneity (Table 4). Heterogeneity factors included species, I/R method, anesthesia, reperfusion time, staining method, RIPC site, duration, and cycles. The results indicated that the study type (p = 0.001) and staining method (p = 0.013) might be sources of heterogeneity.
Factor | Coefficient | Std. Err | t | p value | 95% CI |
---|---|---|---|---|---|
Species | 1.060412 | 0.7194901 | 1.47 | 0.149 | –0.3987814, 2.519606 |
Anesthesia | 0.278913 | 0.6531675 | 0.43 | 0.672 | –1.045772, 1.603598 |
I/R method | –2.80989 | 0.7620935 | –3.69 | 0.001 | –4.355487, –1.264293 |
Reperfusion duration | –0.5447279 | 0.6522578 | –0.84 | 0.409 | –1.867568, 0.7781122 |
RIPC site | –0.0570877 | 0.2852199 | –0.20 | 0.842 | –0.6355406, 0.5213651 |
RIPC duration | –0.2409839 | 0.3198366 | –0.75 | 0.456 | –0.8896425, 0.4076747 |
RIPC cycles | –0.5557973 | 0.5625913 | –0.99 | 0.330 | –1.696785, 0.5851908 |
Staining method | –1.331171 | 0.509424 | –2.61 | 0.013 | –2.36433, –0.298011 |
Abbreviation: RIPC, remote Ischemic preconditioning; I/R, ischemia/reperfusion; CI, confidence interval; Std. Err, standard error. |
The funnel plot was unsymmetric and the Egger’s test (p
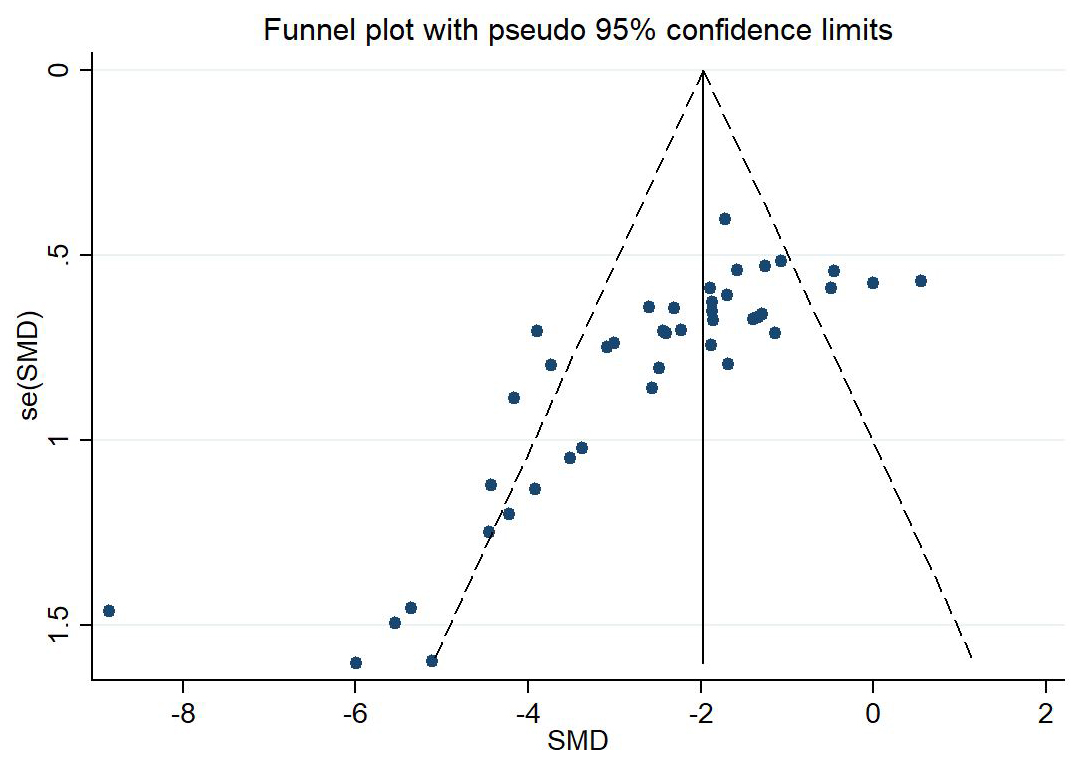
Assessment of publication bias by Funnel plot. The dotted line indicated the standardized mean difference (SMD). The funnel plot was unsymmetric and indicated the existence of publication bias. SMD, standardized mean difference.
The nonparametric trim-and-fill method was applied to adjust the effect size,
and the results were robust (p
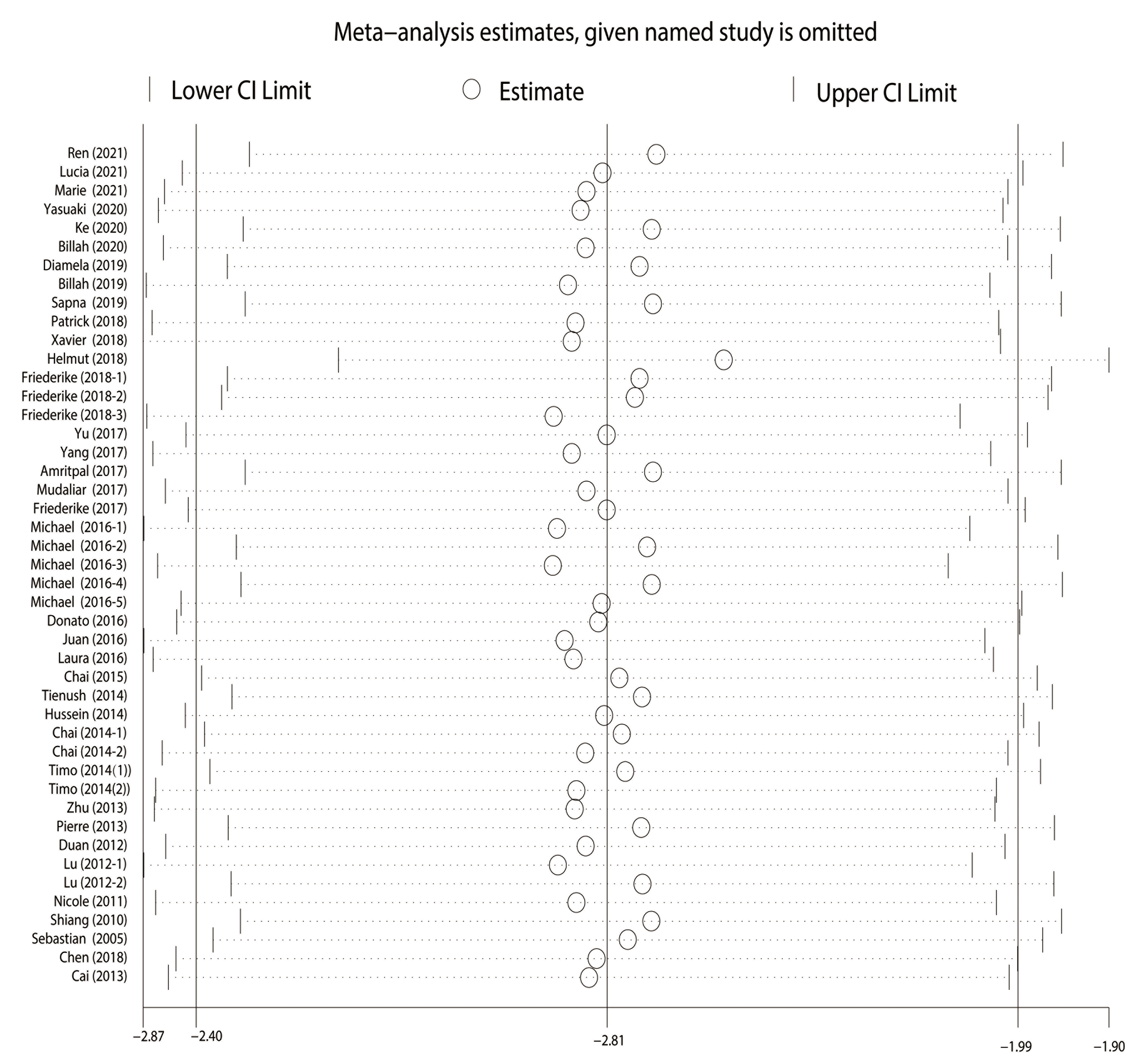
Sensitivity analysis. The round shape represented the estimated pooled effect when a given named study was omitted. The vertical line indicated the lower and upper confidence interval (CI) limit.
The levels of serum cardiac markers, including lactic dehydrogenase (LDH),
creatine kinase-MB (CK-MB), cardiac troponin I (cTNI), and cardiac troponin T
(cTNT), were analyzed (Fig. 5). Studies reporting cTNI levels showed low
heterogeneity (I
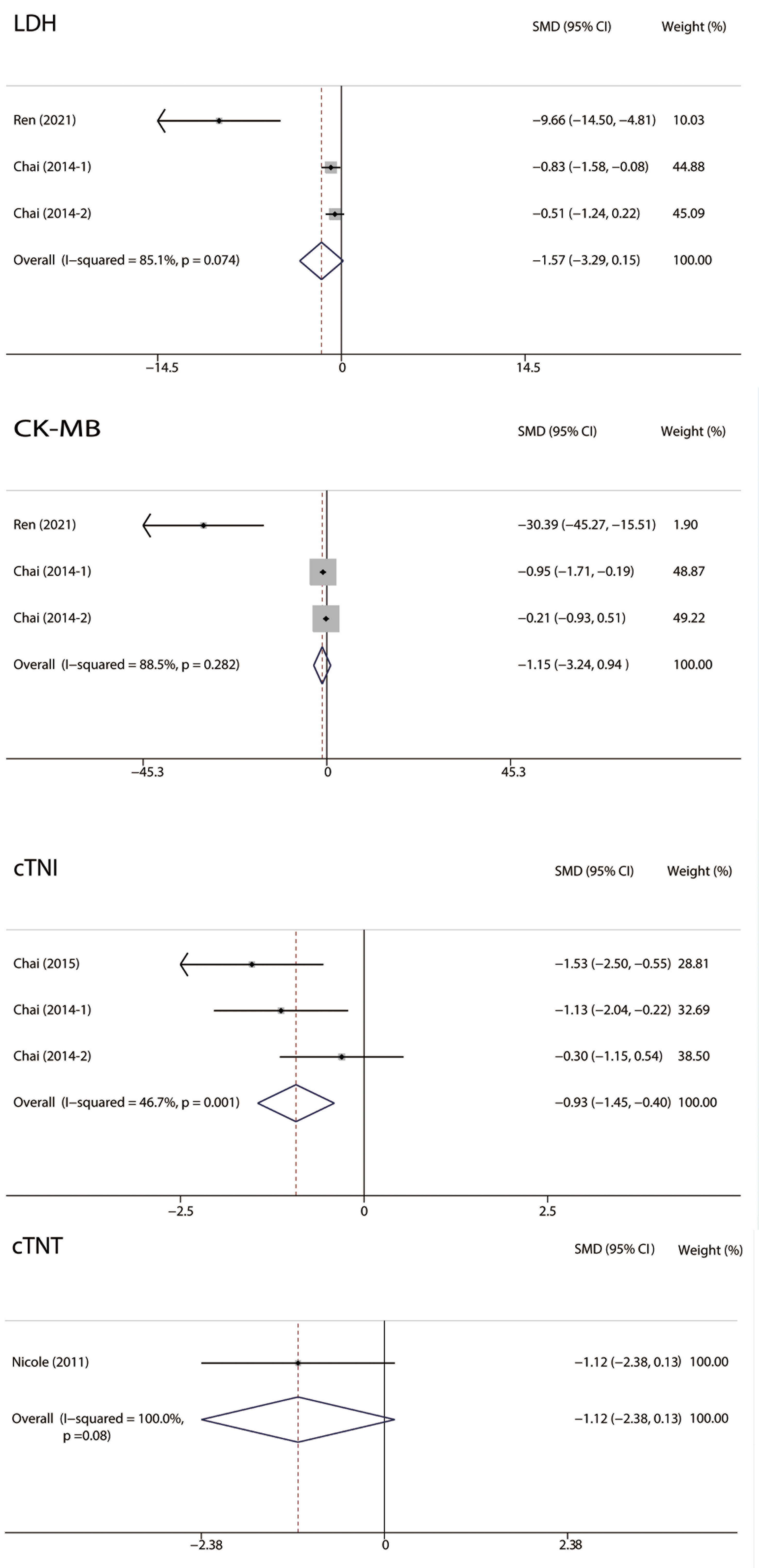
Forest plots of Serum cardiac markers. RIPC was related to significantly lower cTNI levels in MIRI. No significant difference was observed in LDH, CK-MB, and cTNT between the two groups. RIPC, remote Ischemic preconditioning; MIRI, myocardial ischemia-reperfusion injury; CI, confidence interval; CK, creatine kinase; cTNI, cardiac troponin I; cTNT, cardiac troponin T; LDH, lactate dehydrogenase; SMD, standardized mean difference.
As shown in Fig. 6, we analyzed the vital signs of MIRI animals, including heart
rate (HR), mean arterial pressure (MAP), and rate pressure product (RPP). No
significant difference was observed in HR (I
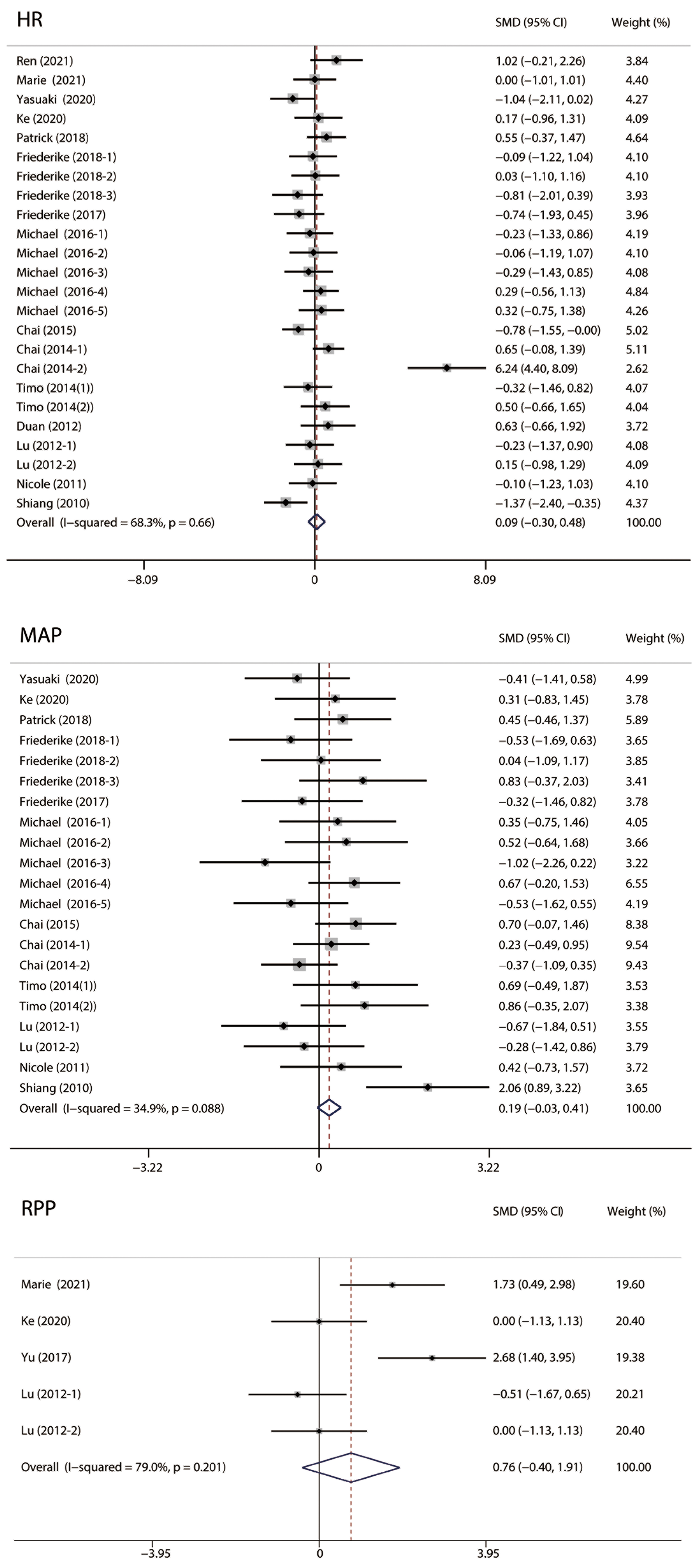
Forest plots of vital signs. No significant difference was observed in HR, MAP and RPP. HR, heart rate; MAP, mean arterial pressure; RPP, heart rate-blood pressure product.
Left ventricular developed pressure (LVDP), recovery of LVDP, left ventricular
systolic pressure (LVSP), left ventricular end-diastolic pressure (LVEDP),
coronary flow (CF), ventricular tachycardia (VT), ventricular fibrillation (VF),
dp/dt max and dp/dt min were included in the comparison of hemodynamic parameters
between the RIPC and control groups (Fig. 7). LVDP (I
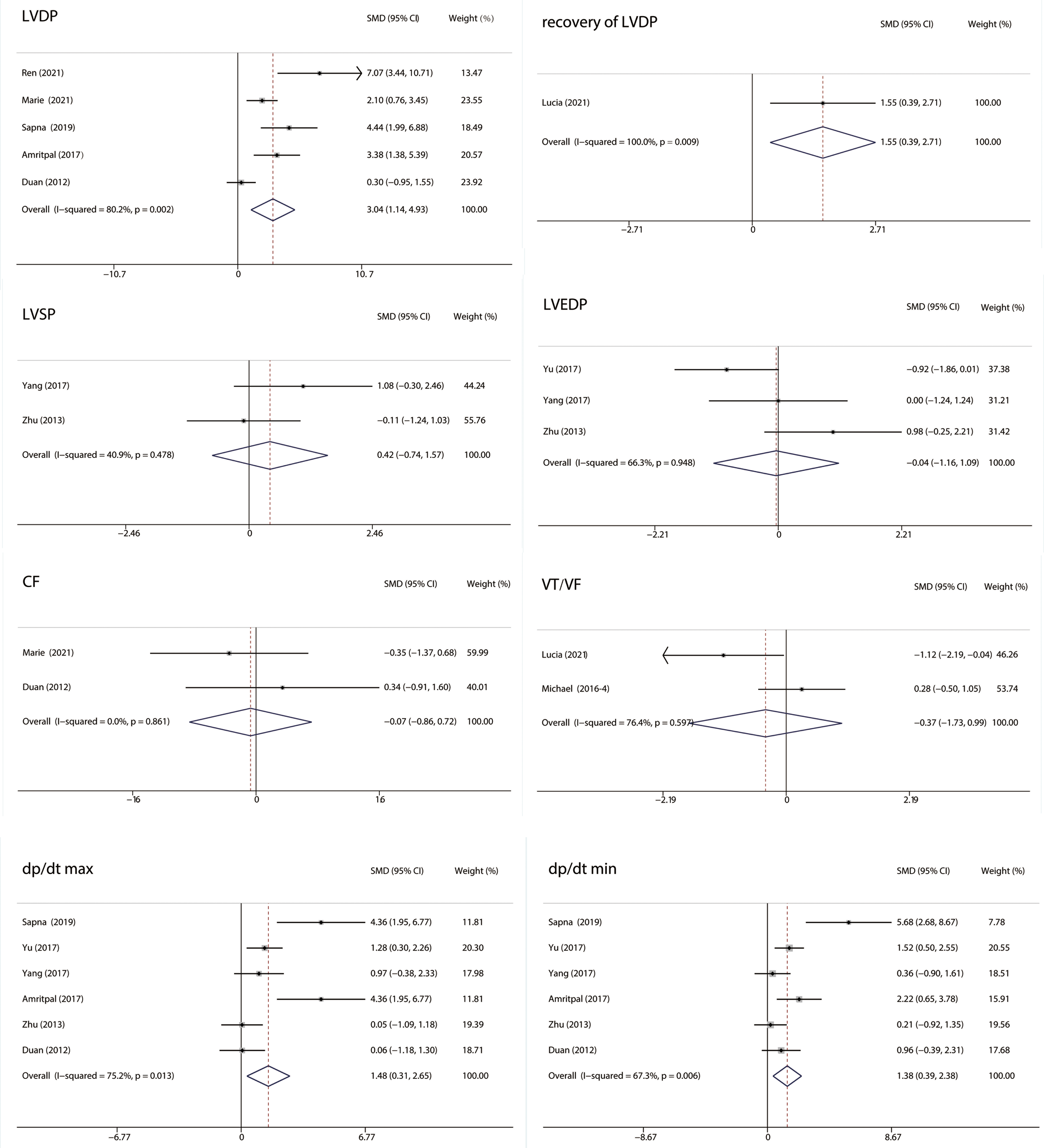
Forest plots of hemodynamic parameters. LVDP, recovery of LVDP, dp/dt max, and dp/dt min were significantly higher in the RIPC group. No significant difference was observed in LVSP, LVEDP, CF, and VT/VF between the two groups. RIPC, remote Ischemic preconditioning; CI, confidence interval; SMD, standardized mean difference; CF, coronary flow; LVDP, left ventricular developed pressure; LVEDP, left ventricular end diastolic pressure; LVSP, left ventricular systolic pressure; VT, ventricular tachycardia; VF, ventricular fibrillation.
Six studies [26, 29, 37, 40, 47] used the terminal deoxynucleotidyl transferase dUTP nick end
labeling (TUNEL) method to identify cell death and no heterogeneity was observed
(I
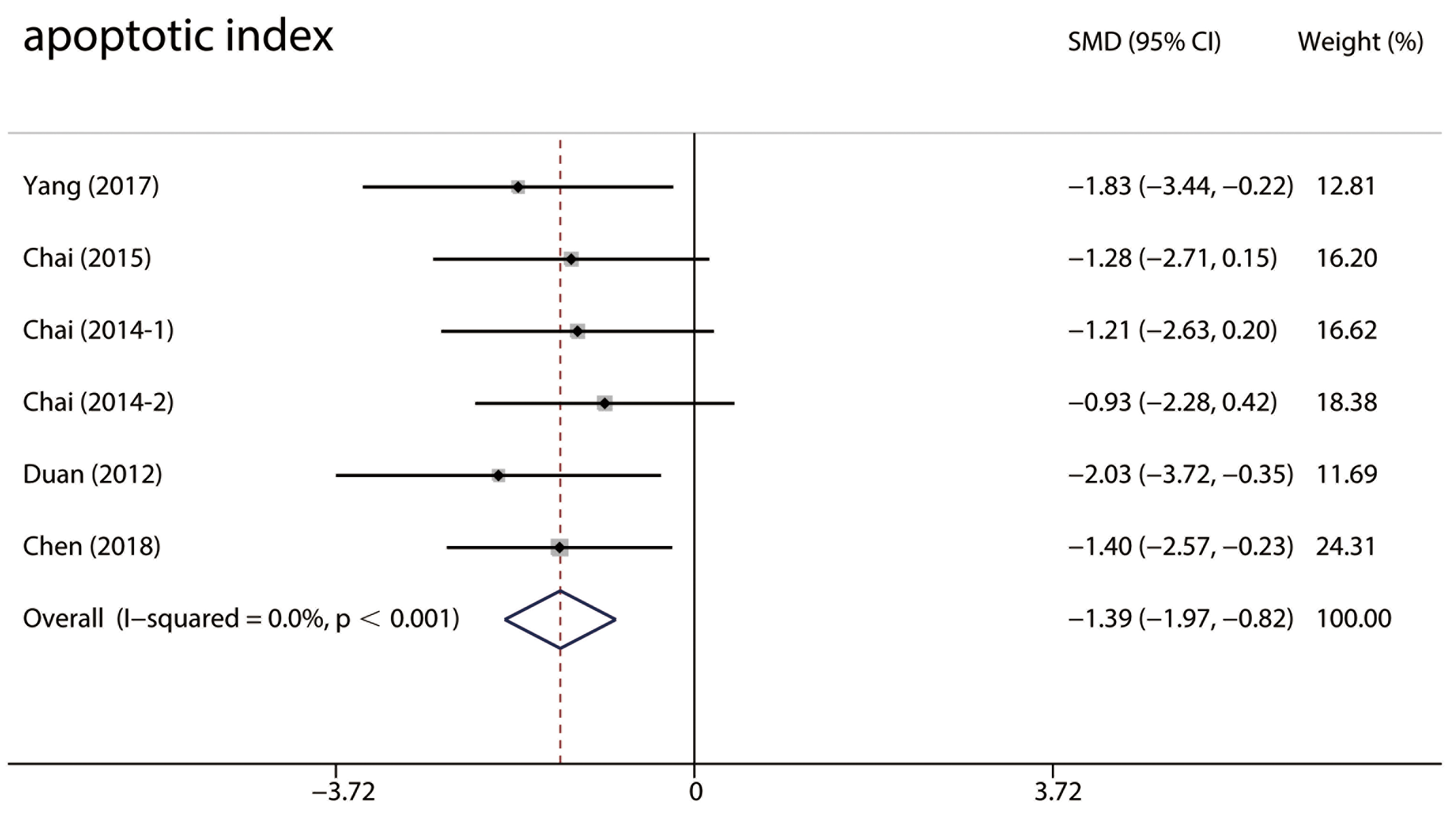
Forest plots of the TUNEL-positive cells. TUNEL-positive cells in the RIPC group were significantly less compared with the control group in MIRI animals. RIPC, remote Ischemic preconditioning; CI, confidence interval; MIRI, myocardial ischemia-reperfusion injury; SMD, standardized mean difference.
This systematic review and meta-analysis included 713 male animals from 37 studies. The infarct size was considered the primary outcome, and all the studies’ data were complete. Forest plots, subgroup analysis, sensitivity analysis, meta-regression, funnel plot, Egger’s test, and Begg’s test were performed. This study found that RIPC significantly protected against MIRI in rats and mice by reducing infarct size, decreasing serum myocardial markers and the number of TUNEL-positive cells, and improving cardiac function. Furthermore, we also provide evidence regarding the factors influencing RIPC, such as the cycles, duration, and site of RIPC, which might be helpful in the clinical setting.
RIPC is expected to benefit the progression of cardiovascular disease as a noninvasive and effective treatment. The mechanism of cardioprotection induced by RIPC contains two phases. The early phase follows the RIPC stimulus and lasts for 1–2 h, while the delayed phase appears 12–24 h later and lasts for 48–72 h [51]. In the early phase, RIPC protects against MIRI via humoral, neuronal, and systemic pathways [12]. The signaling pathways, including the reperfusion injury salvage kinase pathway and the survival activating factor enhancement pathway, are involved in protecting the myocardium [52]. In the delayed phase, RIPC protects against MIRI by downregulating the oxidative and inflammatory injury gene expression, and the mTOR signaling, whereas enhancing the autophagy signaling [12, 53, 54]. Redox stress is related to inflammasomes [55] such as Nlrp3, which can activate caspase-1, damage the cell membrane, cause pyroptosis, and contribute to MIRI [56, 57, 58]. The balance of redox reactions, including that between reactive oxygen and nitrogen species, plays an important role in MIRI and participates in cardioprotection from preconditioning and postconditioning [59] by a specific posttranslational modification (S-nitrosylation of proteins) [60, 61]. In addition, it is emerging that inflammation plays an important role in long-term cardioprotective effects, including cardiac remodeling and heart failure, which has been neglected in previous studies [62].
Primary outcome analysis using Forest plots suggested that RIPC significantly reduced infarct size, similar to that reported in a previous systematic review and meta-analysis that included data from 653 animals and analyzed the effect of remote ischemic conditioning (RIC) in in vivo animal models of MIRI [63]. In the analysis of secondary outcomes, only RIPC significantly reduced the level of cTNI, which might be because cTNI has higher sensitivity and specificity for myocardial injury than LDH, CK-MB, and cTNT [64, 65]. We also found higher LVDP, recovery of LVDP, dp/dt max, and dp/dt min in the RIPC group, representing better left ventricular diastolic function. Another study also suggested that LVEDP and LVDP were associated with reduced infarct size in RIC, which is consistent with our results [54]. In addition, the TUNEL-positive cells were significantly fewer in the RIPC group. However, TUNEL staining may indicate cell apoptosis or necroptosis [66]. The major cell death contributing to MIRI includes apoptosis or necroptosis in non-cardiomyocytes and necroptosis or pyroptosis in cardiomyocytes [5]. Contribution of apoptosis in cardiomyocytes in MIRI is controversial. Inserte et al. [67] suggested that caspase-mediated apoptosis does not significantly contribute to infarct size and ventricular remodeling in MIRI. Therefore, TUNEL-positive cells do not only refer to apoptosis; other cell death mechanisms that contribute to MIRI, such as necroptosis and pyroptosis, also need to be considered in further study. Furthermore, no significant differences in vital signs were observed between the two groups. This might be because a high HR can maintain blood pressure in rats and mice.
In the subgroup analysis, RIPC in the hepatic vessel and infrarenal aorta groups did not show a significant protective effect, indicating that cardioprotection depends on RIPC sites. Different mechanisms, including humoral, neuronal, or systemic pathways, might be related to different organs subjected to RIPC, which could explain this phenomenon [33]. A previous study indicated that the number and duration of RIPC cycles determine the efficacy of the RIPC [68]. This study observed an interesting phenomenon: compared with the same RIPC duration of ischemia and reperfusion (5/5 min, 10/10 min, 15/15 min), RIPC durations of 5/15 min and 30/15 min did not show a significant reduction in infarct size in MIRI. Based on the hypothesis of Galagudza et al. [33], the duration of ischemia and reperfusion might influence the balance between the accumulation of metabolites and/or signaling molecules and the washout of these signaling agents, which influence the triggers of the cardioprotective response in the heart. Therefore, the different durations of ischemia and reperfusion might have disrupted the balance and reduced RIPC cardioprotection in the 5/15 min and 30/15 min groups.
The subgroup analysis and meta-regression results indicated that the possible source of heterogeneity was the I/R method and staining method. The I/R method included in vivo and ex vivo studies; therefore, the systemic response that relies on circulation, such as inflammatory reaction in MIRI, might cause heterogeneity among studies. The staining methods of MIRI included staining with TTC and TTC-Evans blue/patent blue/black Chinese ink, which could lead to different sizes of the area at risk (AAR) and influence the result of infarct size/AAR, resulting in high heterogeneity. As for species, rats have lesser collateral blood flow and faster infarct progression than mice, which might influence the infarct size, but it was not a possible source of heterogeneity in this study [69]. Despite similarities in the cycles and duration, the results can still differ in terms of the efficacy of RIPC in MIRI. In a previous systematic review and meta-analysis, Bromage et al. [63] reported significant heterogeneity that could not be explained by any of the experimental variables analyzed by meta-regression. Recently, Penna et al. [70] reported that keeping the ischemic conditioned limb warm (40 °C) can increase the cardioprotective efficacy of RIPC, indicating that limb temperature could be a potential source of heterogeneity that was not considered in the report by Bromage et al. [63] and this meta-analysis because of missing data.
The funnel plot, Egger’s test, and Begg’s test suggested the existence of publication bias, which may be because some studies did not report negative results. However, the sensitivity analysis and the nonparametric trim-and-fill method showed that the overall effect of RIPC on infarct size in MIRI was robust. The quality of the included studies varied, mainly because of the differences in random housing and blinding methods.
In a multicenter, randomized controlled trial, RIPC did not show a relevant benefit for cardiac surgery, which was different from preliminary experience in animals [8]. The discrepancies between animal studies and clinical studies contains the age of patients and animals, RIPC site, and outcome measures. In this clinical study, the mean age of patients was 65.8 years, the RIPC was induced in upper limbs, and the outcome measures included a composite of death, myocardial infarction, stroke, or acute renal failure. In animal studies, researchers often choose the hind limbs as the RIPC site and measured the infarct size in young mice and rats. The age was considered as one of the operative mortality risk factors [71], which might influence the results. In addition, the nervous system takes part in protective effect of RIPC [25]. Therefore, differences in the neural pathways of the upper and hind limbs may affect the efficacy of RIPC. Furthermore, the outcome measures in clinical study were more complicated than in animal studies, which might lead to controversial results.
This study had several limitations, which should be mentioned. First, evaluation might be influenced by high heterogeneity owing to differences between animal experiments; better-performed studies with particular emphasis on the detailed characterization of RIPC protocols and analysis are warranted. In addition, we did not include studies with female animals because endogenous estrogen was suggested to limit cardiomyocyte apoptosis from MIRI by producing a baseline anti-apoptotic profile; further studies could focus on the potential effect of sex [72]. Moreover, only studies in rats and mice were analyzed. The potential benefits of RIPC in larger species and the presence of comorbidities need to be investigated before studies in humans.
This systematic review and meta-analysis analyzed the protective effect of RIPC against MIRI. It was found that RIPC significantly protected against MIRI in small animal models by reducing infarct size, decreasing serum myocardial markers and limiting cell death, and improving cardiac function. RIPC duration and site influence the protective effect of RIPC on MIRI, which contributes in reducing confounding factors and determines the best approach for human studies.
LC and YW designed this meta-analysis, analyzed the data, and wrote the article. TZ and LY revised the manuscript. JL, PLY and ALQ collected the data for this study. All authors contributed to editorial changes in the manuscript. All authors read and approved the final manuscript.
Not applicable.
Not applicable.
This study was funded by the Science and Technology Department of Sichuan
Province (grant 2022NSFSC0710), Chengdu Science and Technology Bureau (grant
2022-YF05-01343-SN), and 1
The authors declare no conflict of interest.