Academic Editor: Jerome L. Fleg
Noncommunicable chronic diseases, such as obesity, cardiovascular disease (CVD),
and type 2 diabetes (T2D), pose significant health challenges globally. Important
advances have been made in the understanding of the pathophysiologal mechanisms
and treatment of noncommunicable diseases in recent years. Lack of physical
activity is a primary contributor to many noncommunicable diseases including
metabolic syndrome, T2D, CVD, and obesity. Certain diabetes medications and
non-pharmaceutical interventions, such as physical activity and exercise, are
shown to be effective in decreasing the CVD risks associated with heart disease,
stroke, obesity, prediabetes, and T2D. The ability to measure and analyze
circulating adult stem cells (ASCs) has gained particular interest due to their
potential to identify at-risk individuals and implications in various
therapeutics. Therefore, the purpose of this narrative review is to (1) provide
an overview of ASCs; specifically endothelial progenitor cells (EPCs) and
mesenchymal stromal cells (MSCs), (2) describe the responses of these cells to
acute and chronic exercise, and (3) highlight the potential effect of exercise on
EPCs and MSCs in aging and disease. EPCs are circulating cells, abundantly
available in peripheral blood, bone marrow, and umbilical cord, and are defined
by cell surface markers such as CD34
The World Health Organization (WHO) estimates nearly 450 billion people are
suffering from diabetes globally [1]. As per the Centers for Disease Control and
Prevention (CDC), nearly 96 million Americans have prediabetes with more than 37
million having diabetes [2]. Individuals with prediabetes are at high risk for
developing type 2 diabetes (T2D), heart disease, and stroke [2]. The CDC also
estimate that in the United States, 1 out of 3 adults suffer from high blood
pressure, 1 out of every 20 deaths are due to stroke, and 1 out of every 4 deaths
are due to coronary artery disease (CAD) [3, 4, 5]. Overweight and physical
inactivity are two of the leading preventable risk factors for T2D. Being
overweight or obese increases the risk for T2D, with 89.8% being overweight or
having obesity (i.e., body mass index of 25 kg/m
Lack of physical activity is a primary contributor to many noncommunicable diseases including metabolic syndrome, T2D, cardiovascular disease (CVD), and obesity [7, 8, 9, 10]. Robust evidence supports that high amounts of sedentary behavior increase the risk for all-cause and CVD mortality and T2D [11, 12, 13, 14, 15, 16]. For example, an inverse, non-linear dose-response relationship is observed between long-term leisure-time physical activity and all-cause and CVD mortality when assessed during up to 23-years of follow-up [14]. In adults, exercise capacity and energy expenditure are shown to be stronger predictors of all-cause mortality when compared to smoking, hypertension, obesity, and T2D [17]. Recently, Kokkinos et al. [15] showed that cardiorespiratory fitness is inversely associated with mortality in a graded fashion across age, sex, and race in a cohort of 750,302 United States Veterans during a median follow-up of 10.2 years. The lowest risk for mortality was seen at approximately 14 metabolic equivalents (METs) for men and women, with the risk for least fit individuals being 4-folder higher than the extremely fit individuals [15]. In addition to cardiorespiratory fitness, sufficient levels of muscular fitness is also found to have protective effects on all-cause and cancer mortality in healthy middle-aged men, men with hypertension, and patients with heart failure [18]. Importantly, it has been suggested that possessing higher muscular fitness may improve, to some extent, the adverse cardiovascular profile of overweight and obese individuals [18]. Despite the known benefits of engaging in physical activity and exercise, only 1 in 4 adults are estimated to meet the recommended levels of physical activity in the United States [19]. Among 383,928 adults (aged 18–80), only 23.5% were found to meet the physical activity guidelines of combined aerobic activity and muscle-strengthening activity [20]. Those individuals less likely to meet the physical activity guidelines tend to be older, women, current smokers, and have poorer self-rated health and lower education/income [20].
Certain diabetes medications and non-pharmaceutical interventions, such as physical activity and exercise, are shown to be effective in decreasing the CVD risks associated with heart disease, stroke, obesity, prediabetes, and T2D [10, 21, 22]. The United States based study Diabetes Prevention Program (DPP), lifestyle intervention significantly reduced the chances of progressing from prediabetes to T2D and risk of developing CVD [23]. According to the CDC, physical activity not only reduces the risk of developing overt T2D and CVD, but also helps to reduce body weight in individuals with obesity and has even been shown to reduce the risk of developing certain cancers associated with obesity [24]. Physical activity increases life expectancy irrespective of age, ethnicity, body shape or body mass [24, 25]. Compared to those who did not meet the physical activity guidelines, individuals who engaged in muscle-strengthening activities or aerobic activities were found to be at reduced risk of all cause mortality with larger survival benefits found in those engaged in both muscle-strengthening and aerobic activities [26]. Similar patterns were observed for cause specific mortality from CVD, cancer, and chronic lower respiratory tract diseases [26]. Importantly, evidence from a metaepidemiological study of 16 meta-analyses found exercise interventions to have similar benefits of many drug interventions for the secondary prevention of coronary heart disease, rehabilitation after stroke, treatment of heart failure, and prevention of T2D [27].
Currently, diabetes medications only evaluate the hypoglycemic effect of a medication and does not always evaluate its potential to improve endothelial function and regeneration. At best, only surrogates of endothelial function are used rather than evaluating cells of endothelial lineage or even hematopoietic lineage, pre- and post-medication. Historically, the U.S. Food and Drug Administration (FDA) did not require information regarding the effects of diabetes medications on cardiometabolic health although in the last 3–5 years that trend appears to be changing. Similarly, the standard practice for monitoring the effect of exercise in clinical practice is by assessing vascular structure and function using non-invasive measures (i.e., vessel size, blood pressure, flow-mediated dilation) or by analyzing plasma or serum biochemistry focusing on surrogates of endothelial function (i.e., interleukins, high-sensitivity C-reactive protein (hs-CRP), lipid profile). However, it may be more informative to directly study cells, such as adult stem cells (ASCs) of hematopoietic lineage, as these cells may serve as a valuable biomarker to detect and monitor the effect of disease status. Furthermore, the evaluation of ASCs in response to exercise may provide insight into the cellular mechanisms underlying the associated health benefits. Therefore, the purpose of this narrative review is to (1) provide an overview of ASCs; specifically endothelial progenitor cells (EPCs) and mesenchymal stromal cells (MSCs), (2) describe the responses of these cells to acute and chronic exercise, and (3) highlight the potential effects of exercise on EPCs and MSCs in aging and disease populations.
Stem cells are undifferentiated cells which can differentiate into specialized
or specific cell type, tissue or organ. Predominantly, natural or non-genetically
modified stem cells are divided into embryonic and somatic or ASCs. ASCs hold
promise both as a regenerative tool, in a pro-apoptotic (and pro-inflammatory)
state of prediabetes and T2D but also as a biomarker to monitor progression of a
disease process from prediabetes to T2D, at a cellular level. For the purposes of
this review, we will concentrate primarily on two types of ASCs, one is
hematopoietic cells that may be precursor to mature EPCs and MSCs. Peripheral
blood contains approximately 1% of mononuclear cells as EPCs. EPCs are
circulating cells, abundantly available in peripheral blood, bone marrow, and
umbilical cord. EPCs have been defined by cell surface markers such as CD34
MSCs are essential for maintaining tissue and organ homeostasis. MSCs are defined as multipotent heterogeneous cells that can proliferate in vitro as plastic-adherent cells, have fibroblast-like morphology, form colonies in vitro, and can differentiate into ostyeoblasts, adipocytes, chondroblasts, and myoblasts [31, 32, 33, 34, 35, 36]. Sources of obtaining MSC can vary from umbilical cord blood, bone marrow, adipose tissue, pancreatic islet, fetal liver and even the lung tissue [37, 38]. MSCs express CD105, CD73 and CD90 but not CD45, CD34, CD14, or CD11b, CD79a, or CD19 and HLA-DR surface molecules [36]. This is because the latter cell surface markers are thought to be of endothelial cell lineage. The mobilization and homing of MSCs (i.e., the migration and arrest within the vasculature of a tissue followed by transmigration across the endothelium and engraftment in the target tissue) is affected by systemic and inflammatory state [39]. Bone marrow and adipose tissue derived MSCs have been well established for MSC production for therapeutic purposes [31, 32, 34, 40]. For example, MSCs have been used in clinical trials for the treatment of various CVD’s such as ischemic heart disease, cerebro-vascular stroke, chronic kidney disease, and peripheral vascular disease (PAD) [34, 41]. MSCs are also involved in the processes that support skeletal muscle repair in response to resistance exercise [29, 42, 43].
EPCs can act as a cellular biomarker that is more reliable than commonly used clinical serum-based markers for estimating and following endothelial dysfunction in aging, early T2D and prediabetes subjects, pre- and post-exercise, and even in response to medications [44, 45, 46, 47, 48, 49, 50, 51]. Older adults are shown to have lower baseline levels of HSC, EPC, angiogenic T cells, as well as chemokine receptor 4 expressing circulating angiogenic cells (CXCR4-expressing CACs) [52, 53, 54, 55]. Similarly, baseline counts of hematopoietic stem cells (HSCs), EPCs, and expression of CXCR4 and CXCR7 were significantly lower at rest in T1D group compared to healthy control group [56, 57]. Older adults demonstrated endothelium-dependent dilation of the brachial artery when compared to young healthy individuals. While there was no differences in the number of circulating EPCs; lower survival, migration, and proliferation was observed suggesting functional impairment of EPCs in older adults [58].
The level of circulating CD34
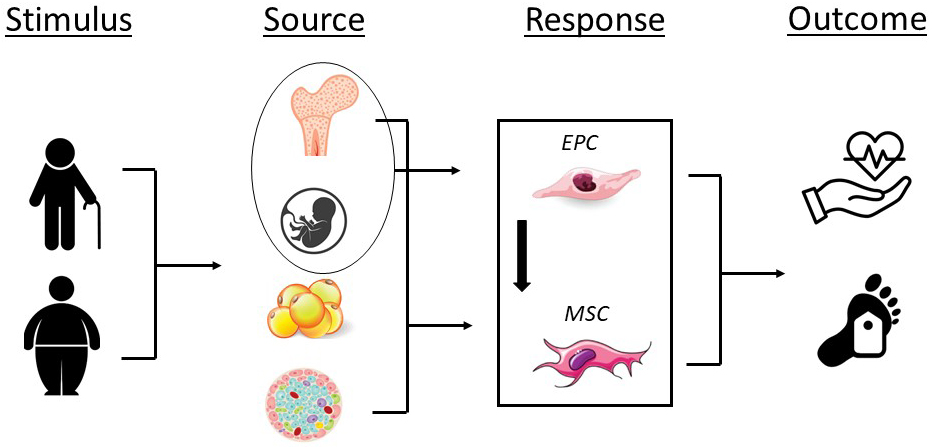
Impact of aging, obesity, and disease on endothelial progenitor (EPCs) and mesenchymal stromal cell number (MSCs) and/or function and the potential for adverse cardiovascular events and all-cause mortality. Stimulus; aging, obesity; Source, bone marrow, umbilical cord blood, adipose tissue, pancreatic islet; Response, decrease in EPC and MSC number and/or function; Outcome, adverse cardiovascular events, all-cause mortality.
Physical activity and exercise are essential for the maintenance and improvement of health and function [24, 25, 26]. Physical activity is defined as any bodily movement produced by skeletal muscles that results in an increase in caloric requirements above resting energy expenditure [67]. Exercise refers to a specific type of physical activity consisting of planned, structured, and repetitive bodily movement to improve and or maintain one or more components of physical fitness [67]. Aerobic activities, which consist of repetitive rhythmic movements such as walking, running, cycling, and swimming, are most often prescribed for targeting cardiovascular health. Muscle-strengthening activities (i.e., resistance exercise) include weight machines, free weights, resistance bands, and body-weight exercise and are prescribed for neuromuscular health. For older adults and adults living with chronic diseases, engaging in at least 150 minutes (2 hours and 30 minutes) to 300 minutes (5 hours) per week of moderate-intensity, or 75 minutes (1 hour and 15 minutes) to 150 minutes (2 hours and 30 minutes) a week of vigorous-intensity aerobic physical activity, or an equivalent combination of moderate- and vigorous-intensity aerobic physical activity is recommended [19, 67]. In addition, adults are encouraged to combine aerobic activity with 2 or more days per week of muscle-strengthening activities of moderate or greater intensity involving all major muscle groups [19, 67]. While meeting the physical activity and exercise recommendations are preferred, simply increasing the amount of physical activity and exercise above sedentary levels will confer health benefits even if the recommended guidelines are not achieved [68, 69]. Despite the importance of physical activity and exercise for maintaining or improving overall health, limited data exists on the effects of physical activity and exercise on EPCs and MSCs.
Studies investigating the responses of EPCs and MSCs show transient increases in cell numbers in response to exercise (Fig. 2) [29, 70, 71, 72]. Ferentinos et al. [70] conducted a systematic review and meta-analysis examining acute effects of different forms of exercise on circulating EPCs in healthy populations and found that prolonged endurance and resistance exercise had the most profound effect on circulating EPCs followed by maximal exercise. In another systematic review and meta-analysis examining healthy adults it was demonstrated that the extent and time-course of exercise-induced mobilization of circulating stem and progenitor cells differed between stem cell-subpopulations; i.e., EPCs, HSCs, and MSCs [71]. Early and non-specified stem cells were shown to increase significantly immediately after the initiation of exercise (i.e., 0–5 minutes) until 30-minutes post-exercise [71]. For EPC numbers, a significant increase was found until 12-48 hours after exercise and for HSC numbers at 0-5 minutes and at 3 hours after exercise [71]. No effect of exercise on MSC numbers was observed [71]. Importantly, these findings were not influenced by sex, intensity, or duration of the interventions assessed [71].
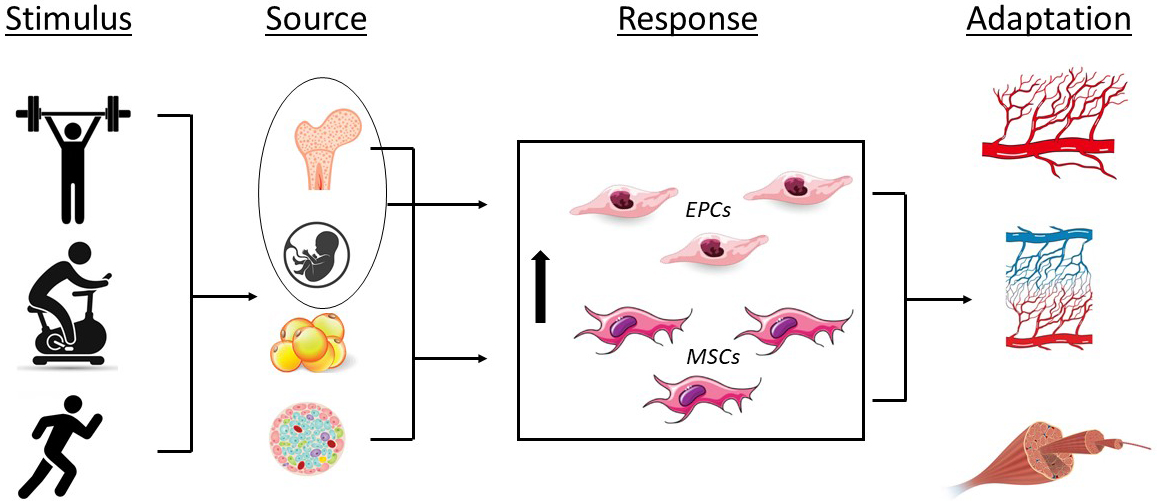
Effects of exercise on endothelial progenitor (EPCs) and mesenchymal stromal cells (MSCs) and the associated physiological adaptations. Stimulus; resistance exercise, cycling, running; Source, bone marrow, umbilical cord blood, adipose tissue, pancreatic islet; Response, increase in EPC and MSC number and/or function; Adaptation, angiogenesis, capillarization, skeletal muscle tissue repair.
In young healthy trained men, circulating EPC and serum concentrations of
vascular endothelial growth factors (VEGF-A, VEGF-C, and VEGF-D), granulocyte
colony stimulating factor, soluble Tie-2, soluble fms-like tyrosine kinase-1, and
matrix metalloproteinases (MMP-1, MMP-2, MMP-3, MMP-9, and MMP-10) were higher in
the postexercise period following a muscular endurance resistance exercise
program (three circuits of 15-repetitions of six exercises) [73]. Circulating EPC
were unchanged at 10-minutes postexercise but higher at 2-hours postexercise while
the concentration of most angiogenic factors and metalloproteinases were higher
at 10-minutes postexercise [73]. In young healthy women, resistance exercise using
intensities of 60%, 70% and 80% 1-repetition maximum performed for 3 sets of
12-repetitions increased circulating EPC and levels of VEGF, hypoxia-inducible
factor 1-alpha (HIF-1
Moderate levels of physical activity (50–70% of maximal heart rate for 20-minutes daily for 5 days per week) increase circulating numbers of EPCs and increases EPC colony count formation which appears to have an anti-apoptotic effect in subjects with pre-diabetes [75]. Such an effect of EPC is likely to depend on the degree of inflammation, hyperglycemia, and intrinsic antioxidant enzyme presence in the EPC. Similarly, it is also reported that exercise helps to reduce apoptosis that is mediated by phosphatidylinositol 3 (PI3)-kinase pathway which is dependent on nitric oxide bioavailability [76]. Prostaglandin E1 (PGE1)-mediated upregulation of EPC is also linked to the improvement of EPC function and improved angiogenesis [77]. The improvement of EPC number may be related to, and even preceded by, an increase in plasma VEGF [44]. For example, in patients with CAD, exercise induces a short-term cellular ischemia which increases HIF which in turn increases EPC number (dependent on VEGF and HIF) [44].
Exercise-induced osteogenesis are observed for bone marrow-derived MSCs [78]. Data from our laboratoryrevealed that exercise promotes osteogenic differentiation of fat derived MSCs in prediabeteic Veteran’s [79]. Interestingly, bone differentiation markers including RUNX genes, alkaline phosphatase (ALPL), and osteocalcin were significantly upregulated indicating osteogenic differentiation [79]. Cook et al. [80] discussed how signaling pathways manipulate MSC differentiation. Both bone morphogenic protein (BMP) and WNT signaling pathways play an important role in MSC differentiation towards bone formation following exercise. WNT signaling promotes osteogenic differentiation by upregulating RUNX (an important gene associated with bone formation) and by inhibiting peroxisome proliferation-activated receptor gamma (PPARᵞ) (a gene that promotes adipogenesis). On the other hand, BMPs activate osteogenic differentiation by activating a prominent bone forming transcription factor, RUNX2 [80]. Maredziak et al. [81] also showed that four-week old male C57B1/6 mice, 5-weeks of treadmill exercise increases bone marrow derived MSC number. Markers associated with osteogenesis (i.e., ALPL activity, osteopontin, and osteocalcin) were also found to be increased postexercise [81].
Collectively, exercise facilitates EPC differentiation and production from bone
marrow and also helps to promote migration and homing-in of the progenitor cells
to the hypoxic and damaged tissue which in turn improve angiogenesis and
vasculogenesis [82]. As might be expected, the observed responses of increased
count following exercise wane over time. Duration of exercise undertaken on a
daily basis influences circulating EPCs numbers [44]. It has been reported that
intensive and moderate exercise activity for 30-minutes increases circulating EPC
number. However, this outcome is not seen when the time of exercise is reduced to
10 minutes [45]. It has also been demonstrated that a maximal bout of exercise
stimulates a significant shift in CD34
In older adults and clinical populations, physical activity and exercise elicit
beneficials effects on endothelial function which may be explained, in part, by
responses of EPCs and MSCs [83, 84]. Physical activity was associated with
CD34
Intravascular ultrasound-based studies have shown atherosclerotic plaque
reduction or retraction in response to exercise [87]. It has been reported that
supervised exercise sessions boost the circulating EPC counts while increasing
angiogenesis and improved endothelial function thereby decreasing the incidence
of atherosclerosis [88, 89, 90]. In patients with hypertension, exercise reduces
dysfunction of EPC, which promotes neovascularization and improves hypertension
[91]. Mechanical stress to the tissue and vasculature is posited as a primary
mechanism underlying the promotion of enhanced EPC function following exercise
[82]. The mechanical force resulting from exercise directly or indirectly helps
in improvement of EPC number and function [82]. Chronic moderate-intensity
continuous exercise is shown to have a positive effect on circulating EPCs in
older sedentary individuals which was accompanied by improvements in endothelial
function and arterial stiffness [70]. In response to 8-weeks of cycling exercise
at 65–85% heart rate reserve (HRR), there was no effect on baseline or
exercise-induced numbers of HSCs and EPCs [54, 92]. Habitual physical activity in
patients with CAD is associated with higher flow-mediated dilation and EPC count
[93]. However, flow-mediated dilation was only related to increased habitual
physical activity levels but not EPC count [93]. Patients with type I diabetes
(T1D) performed 45-minutes of incline walking at 60% maximal oxygen consumption
(VO
Studies have showed that, similar to observations of EPCs, exercise may facilitate MSC homing into the site of injury [94]. It was reported that exercise induce homing of MSCs to extramedullary sites [95]. Often, the effect of MSC integration with host tissue and subsequent repair, increase with exercise [96]. It has also been reported that exercise increased the efficiency of MSC transplantation in cerebral ischemia in rats by inhibiting apoptosis [97]. Another study showed stromal vascular fraction, a well-known mixed population enriched with MSCs, when combined with exercise, together, help to improve pain in patients with knee osteoarthritis (OA) thereby establishing the synergistic effect of cell therapy and exercise in healing a common joint problem such as OA [98, 99]. Exercise also plays a vital role in differentiation of multipotent MSCs towards various adult tissues. MSCs can differentiate into bone, muscle, cartilage and adipose tissue depending on the need of the body’s reparative process. The differentiation is also dependent on the cellular environment.
The use of ASCs as a means of assessing and monitor health status is an evolving area and shows promise for the advancement of clinical care. However, several questions remain before its application can truly be appreciated. The lack of standardized designations of cells creates challenges for comparing findings across different research laboratories. The definition of EPC need to carefully delineated based on cell surface markers rather than cell type using phenotypic nomenclatures. Following appropriate cell surface-based designations, functional assays and there related reviews, can then be focused on. While increases in circulating ASCs are seen in response to both aerobic and resistance exercise, the exact dosing of such interventions has yet to be determined. Moreover, the time course of local and systemic adaptations associated with ASC response to exercise in aging and clinical populations requires further investigation. Additionally, given that most patients are prescribe one or more medications depending on their existing conditions, it will be essential to determine how ASCs respond to exercise interventions in the presence of specific medications.
ASCs, such as EPCs and MSCs, can act as a cellular biomarker for cardiovascular diseases, metabolic diseases, chronic rheumatological diseases, and infectious diseases. Use of ASCs as a biomarker have the potential to revolutionize the management of patients with a variety of metabolic and obesity related disorders and also pro-inflammatory diseases. Exercise offers beneficial effects on the proliferation and migratory function of EPCs and MSCs. Further investigation of clinical entities are urgently needed to understand the implications of interventions such as exercise, diet, and various medications on EPC and MSC quantity and function in aging and clinical populations.
NIDDK, National Institute of Diabetes and Digestive and Kidney Diseases; WHO,
World Health Organization; CDC; Centers for Disease Control and Prevention; CAD,
coronary artery disease; T2D, type 2 diabetes; CVD, cardiovascular disease; METs,
metabolic equivalence; hs-CRP, high-sensativity C-reactive protein; FDA, U.S.
Food and Drug Administration; ASC, adult stem cells; HSC, hematopoietic stem
cells; EPC, endothelial progenitor cell; MSC, mesenchymal stromal cell;
KDR
SS proposed the conceptualization of the research study. SS and JMG performed the literature review. SS and JMG synthesized the existing data. SS and JMG wrote the manuscript draft. SS and JMG contributed to editorial changes in the manuscript. SS and JMG read and approved the final manuscript.
Not applicable.
Not applicable.
This work was partially supported by the Department of Veterans Affairs Office of Research & Development, Rehabilitation Research & Development Career Development Award (CDA-2; 1IK2RX003423-01) (JMG).
The authors declare no conflict of interest.
Publisher’s Note: IMR Press stays neutral with regard to jurisdictional claims in published maps and institutional affiliations.