Academic Editor: Johannes Ott
Objective: To review the current knowledge on uterus bioengineering and discuss potential future directives. Uterus bioengineering may solve two major hurdles in organ transplantation of a uterus, organ shortage and control of rejection by immunosuppression. Mechanism: Literature search using PubMed. Findings in brief: Sixty-seven references were summarized that describe the scientific progress made on uterus bioengineering, including other studies related to the topic. Most articles describe work on rat models, including proof-of-concept that uterus bioengineering can be used to restore fertility after a partial uterine injury. These promising results are currently being translated to larger and more clinically relevant animal models. In particular, uterus-specific scaffolds produced by a process called “decellularization” that were developed for the mouse, rat, rabbit, pig, goat, and sheep. These scaffolds stimulated angiogenesis and regeneration in vitro and in vivo, and successfully harbored various types of cells for an extended time in vitro. Additionally, applications for endometrial extracellular matrix-specific hydrogels derived from decellularized uterus tissue is discussed. Current challenges for uterus bioengineering are also addressed, e.g., the cellular reconstruction phase, and how they might be improved. Conclusions: Significant progress was made during the last decade with convincing evidence from multiple independent groups in experiments with small animal models. Initial steps towards large animal uterus bioengineering were made. The future continuation of such studies will provide important data required to translate these ideas to an experimental phase in the human. Partial uterus reconstruction through a bioengineered tissue transplantation is closer to a clinical reality compared to whole uterus bioengineering principles aimed to replace a donor in a UTx setting.
Uterus transplantation (UTx) face similar challenges as many other organs used for transplantation with strict donor criteria and limited availability of donor organs. In addition, the recipient will require a carefully composed administration of immunosuppressants to prevent organ rejection. Unfortunately, these drugs may cause adverse side-effects, including increased susceptibility to infections, nephrotoxicity, diabetes and certain malignancies. Therefore, organ bioengineering has received much interest with the transplantation community lately. Its objective is to bypass the hurdles of organ shortage and need for immunosuppression by using a biomaterial (also called scaffold) that can be colonized by the patient’s own cells to generate a patient-specific grafting material. Such bioengineered graft is independent of a specific donor source and could eliminate immunological barriers such as the human leukocyte antigen compatibility and the necessary immunological donor-recipient matching. Hence, a bioengineered grafting material may be used for essential or elective transplantation surgeries to improve quality of life of the patient without the need for immunosuppressive therapy.
The scaffold used in bioengineering should provide structural benefits for the added cells so that an appropriate construct can match the therapeutic requirements. A scaffold production technique called “decellularization” has received much attention in the last decade and will be discussed extensively in this review. Although, the idea of using decellularized tissue for regenerative medicine applications was suggested much earlier, e.g., for nervous system repair [1], it is during the last decade that this technique received considerable attention. Highly cited publications in esteemed journals included the first perfusion protocols on how to decellularize whole rat organs such as the heart, kidney, lung and liver, that presented ideas on how these organ-specific scaffolds could be “recellularized” with various types of cells [2, 3, 4, 5, 6]. Even if these studies presented reconstructed tissue with very rudimentary signs of organ specificity, the potential of using decellularized tissue for organ bioengineering was convincing. When an organ is decellularized, the remaining biological scaffold is optimally free from its immunoreactive cellular components, including donor DNA, while leaving the tissue-specific extracellular matrix (ECM) preserved in its correct 3-dimensional (3D) architecture with patent vascular conduits. These conduits may be used as an infusion port for patient-specific cells in the recellularization process, and for the vascular anastomosis during transplantation. Since there is a close genetic homology for ECM molecules between species, it is generally believed that this type of scaffold is immunologically inert. Thus, even if a donor is required to produce the scaffold, the organ will not need to be tissue matched since donor cells are removed. Additionally, the donor organ may be harvested several hours after circulatory death. Hence, transplantation problems related to donor organ availability and subsequent immunotherapy after transplantation could potentially be bypassed using decellularized tissue as scaffolds.
In the clinic, bioengineered constructs based on both synthetic or biological scaffolds have been particularly successful in bone, skin and vascular applications. However, more complex organ and tissue grafts are challenging to engineer. Hence, this is therefore a rapidly evolving major research topic that unite several large research disciplines, including regenerative medicine, stem cells, cell biology, biomaterials, and transplantation.
Decellularized tissue may not only benefit novel Utx protocols, but may also become advantageous for fertility preservation in young pre-pubertal hematologic cancer patients which are not suited for an ovarian cortex transplantation [7, 8, 9, 10, 11, 12, 13, 14], or to stabilize the pelvic region to cure severe pelvic organ prolapse without the erosion problems associated with current meshes [15, 16]. Furthermore, neovaginas have been constructed for patients with vaginal aplasia [17], and strategies for oviductal reconstruction was also reported [18]. These bioengineering applications show great promises, however, are outside the scope of this review. Instead, this review specifically focuses on the major advancements made in uterus bioengineering.
Some of the pioneering uterus bioengineering studies were developed to establish a more realistic endometrial and myometrial 3D tissue environment in vitro. These cell culture platforms were used to study endometrial cancer invasion mechanisms [19, 20], cellular interactions between stroma and epithelial compartments and embryo implantation [21]. As for many other types of tissues, initial biomaterials were based on collagen, Matrigel® or agar, often applied in different layers together with uterus cells of either rodent, rabbit or human origin [19, 22, 23, 24, 25, 26, 27].
However, more recently, uterus bioengineering research has been focused towards applications for reconstructive surgery to treat various conditions of infertility (Fig. 1). Uterus bioengineering may, e.g., be used to reduce major scarring to the uterus as a consequence from repeated surgeries, or towards other types of interventions that reduce fertility or increase the risk for uterine rupture during a pregnancy. A small bioengineered uterus patch may, in these cases, be inserted at the incision site at the end of the operation to improve and accelerate the healing process and to reduce scarring formation. Alternatively, scarred uterus tissue could potentially be replaced with a bioengineered tissue patch to restore the fertility for the patient. Importantly, these clinical applications serve as an excellent intermediate objective for the more challenging prospect of developing a whole bioengineered uterus that can replace a donor in a UTx setting.
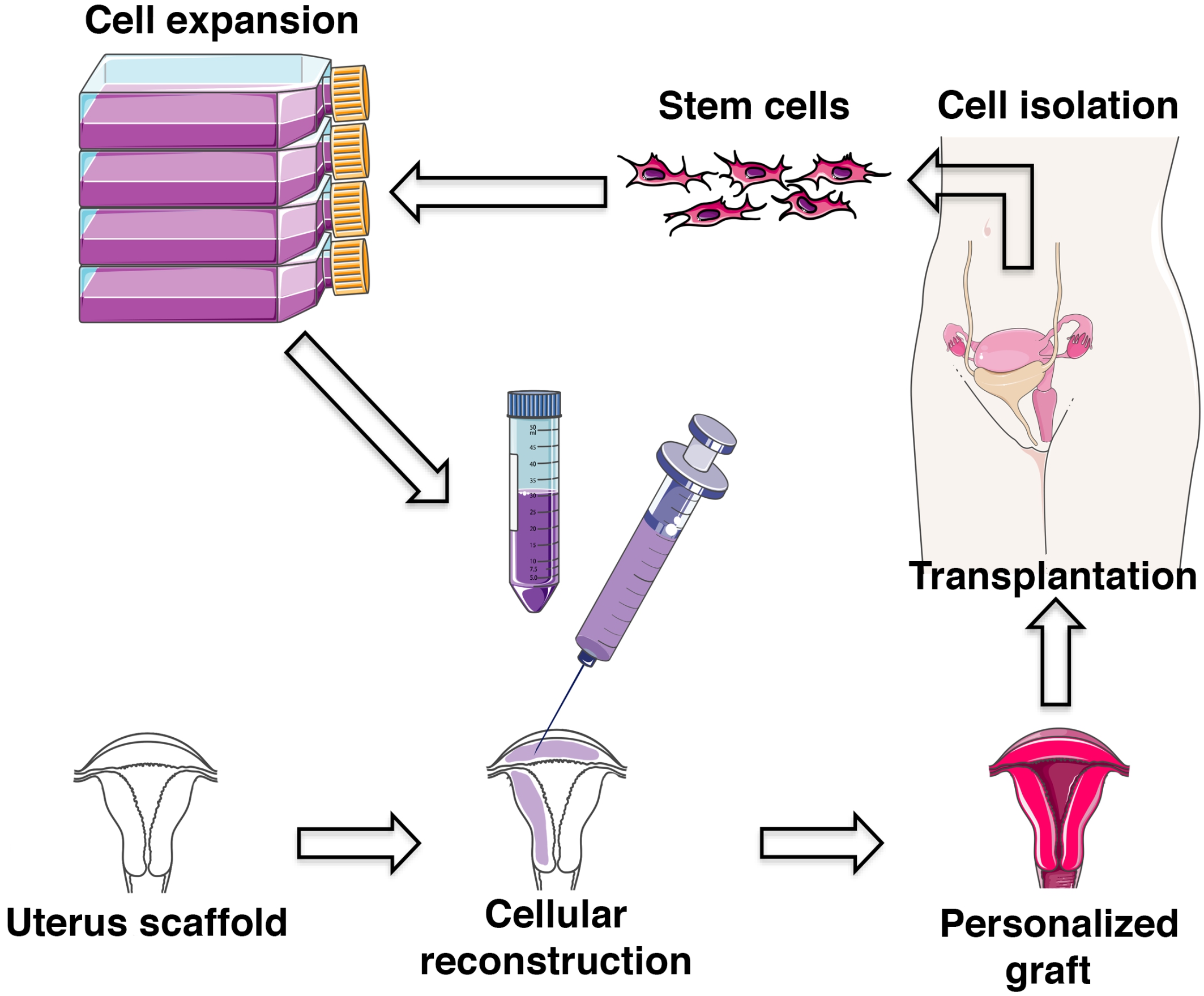
Principles of uterus bioengineering (modified from Servier Medical Art, licensed under a creative common attribution 3.0 generic license; http://smart.servier.com).
The majority of all published papers on uterus bioengineering are based on experiments in rodent models, particularly rats. An early study evaluated if autologous tubular-shaped myofibroblast tissue could be used to replace a large uterine segment in the rat uterus [28]. After 12 weeks, the graft morphology was composed of uterus tissue layers, but were less organized compared with normal tissue. When grafted animals were mated, the operated uterus was able to support pregnancy to full-term. A different group used a similar model to evaluate grafts created from collagen loaded with basic fibroblast growth factor or with bone marrow-derived mesenchymal stem cells (BM-MSCs). These transplants were evaluated after 30 and 90 days. Similar to Campbell et al. [28], they reported successful pregnancy in the operated uterine horn [29, 30].
Interestingly, in 2014, three rat uterus bioengineering studies were published
that used the scaffold production technique called “decellularization” [31, 32, 33]. Miyazaki and Maruyama’s decellularization protocol was based on the rat
liver protocol published by Uygun et al. [5], which proved efficient to
also decellularize a whole rat uterus. As with the liver study, short term
assessment showed that the scaffold remained patent after whole organ
transplantation and vascular anastomosis. The uterine scaffolds were
recellularized with primary uterine cells and MSCs, and a small bioengineered
uterus segment (15 mm
Interestingly, while the orientation of the uterine graft seems to play an important role to achieve morphologically analogous tissue regeneration, the pregnancy results were unchanged when grafts were transplanted in the opposite orientation [35]. The same study also showed that grafts made from decellularized small intestinal tissue were not as advantageous as grafts derived from decellularized uterus tissue. Hence, important tissue-specific regenerative factors seem to remain in the scaffolds after decellularization. Based on a mouse model, an important cellular pathway for uterus regeneration seems to be through the signal transducer and activator of transcription 3 (STAT3) pathway, and that the regeneration is less dependent on the ovarian hormones [36].
One recent publication used a grafting material based on SDS-based
decellularized rat uterus tissue that were recellularized with GFP-labelled human
MSCs [37]. Similar to the results presented in Hellström et al.
[34], few GFP-labelled cells survived more than seven days after engraftment.
Yet, grafts with the cells performed much better than grafts without the MSCs.
The recellularized constructs were also able to restore fertility when the 10 mm
Surprisingly few bioengineering studies using decellularized tissue investigated the immunological response after transplantation, independent of organ or tissue type. However, two recent studies showed that the type of uterus decellularization method can affect the immunogenicity of the scaffolds since immunoreactive molecules known as damage associated molecular patterns (DAMPs) are formed during the decellularization process [39, 40]. DAMPs include fragmented DNA, RNA, cellular and extracellular proteins that become immune-reactive in both an autologous and an allogenic setting. These two studies suggest that a milder decellularization protocol is more favorable from an immunological perspective, and that the donor DNA content in uterine scaffolds should be less than 1% of its original content. However, further studies will be necessary to fully elucidate the immune response after transplantation of decellularized tissue so that bioengineering principles can safely be translated to the clinic. In particular, when scaffolds are combined with commonly used immune modulating cell types (e.g., MSCs).
The rabbit uterus is slightly larger than the rat uterus which simplifies transplantation procedures and enables larger uterus injury-and-repair models. Campo et al. [41], established a perfusion protocol to decellularize whole rabbit uterus with SDS, Triton X-100 and DNase. Rather than using the decellularized tissue as a scaffold for uterus repair, they investigated if the decellularized endometrium could be used to create a hydrogel suitable as a substrate for in vitro embryo culture [41]. Interestingly, they found that hormone treated rabbit donors yielded a more favorable endometrial hydrogel substrate for in vitro rabbit embryo development compared with non-stimulated donors. Their additional proteomic analysis of the produced hydrogels suggested that hormone stimulation prior to uterus explantation resulted in an advantageous molecular scaffold content after decellularization.
In a different study, decellularized rabbit uterus tissue was crosslinked with naturally derived genipin or procyanidins in an attempt to delay the rather quick degradation time of decellularized tissue in vivo [42]. They found that crosslinking the rabbit uterus scaffolds with 0.625% procyanidin significantly delayed scaffold degradation time and improved uterus regeneration when transplanted in a rat xenotransplantation model. However, a few disadvantageous results included a higher proinflammatory response and an elevated infiltration of immune cells with the crosslinked decellularized tissue. If this negative response can be modulated, crosslinking seems a promising approach to improve the mechanical strength and increase the longevity of scaffolds derived from decellularized tissue and accommodate the long healing process required to repair a large uterus injury in vivo.
A mechanically robust scaffold may be one of the principal benefits behind the
good results from a different type of rabbit uterus biomaterial evaluated.
Magalhaes et al. [43] used a bioengineered uterus graft created by a
biodegradable polyglycolic acid (PGA) and poly-DL-lactide-coglycolide (PLGA)
polymer scaffold. They molded the scaffold into a U-shaped 6–8 cm
As mentioned above, several independent research groups have repeatedly shown promising bioengineering strategies for uterine repair in small animal models. In our opinion, these principles are ready to be translated and evaluated in more clinically relevant animal models.
In 2017, it was shown that the pig uterus could be decellularized with a similar
perfusion protocol developed for the rat liver, using the detergents SDS and
Triton X-100 [44]. Histological analysis showed that the intracellular components
were effectively removed while the extracellular matrix composition (collagen,
elastin, fibronectin, laminin and glycosaminoglycans) was preserved. They also
observed a relatively intact sub epithelial capillary plexus with intact conduits
of around 7.5
Recently, a tissue-specific hydrogel was created from decellularized pig uterus endometrium that were used as a 3D substrate to culture human endometrial organoids [45]. The results clearly showed that the organoids exposed to the endometrial ECM-specific hydrogel improved spheroid expansion and cell proliferation compared with standard culture conditions. This is another example how bioengineering principles can provide novel in vitro platforms that better mimic the native 3D environment.
However, the versatility of the same type of pig endometrium ECM-specific hydrogel was demonstrated in a mouse model for Asherman’s syndrome/endometrial atrophy [46]. In this study, the hydrogel was injected into the uterine lumen as a therapeutic substrate, and when it was further enriched with pro-regenerative growth factors, its regenerative effects included improved endometrial gland formation, angiogenesis, and the therapy resulted in higher pregnancy rates.
A pilot study on goat uterus evaluated if the mild detergent protocols developed for rat uterus decellularization could be translated to a slightly larger uterus model [47]. The authors confirmed that both SDC-based, and Triton X-100-based protocols were able to successfully create donor DNA-free goat uterus scaffolds with preserved vascular conduits and extracellular constituents.
Earlier experiences from UTx research showed that the sheep is a good large animal model because of its close resemblance to the human uterus in size, pregnancy characteristics and vascular anatomy [48, 49]. Furthermore, protocols exist for isolating sheep uterus stem cell-like cells [15, 16]. Similar uterus stroma/stem cells are also found in the human uterus [50, 51, 52] that may become a valuable cell source for future uterus bioengineering studies.
For these reasons, a number of different sheep uterine scaffolds have been produced by various methods of decellularization. For example, Daryabari et al. [53] reported three different strategies on how to remove all cells in the sheep uterus using a mild detergent (Triton X-100) or a strong detergent (SDS), respectively. Results from histological analysis and extracellular matrix quantification concluded that their SDS based protocol in combination with a formalin fixation step was the best. Segments of this scaffold type was transplanted into rats in a xenograft study. Infiltration of cells were observed 10 days post transplantation that were positively stained for cluster of differentiation (CD) 31, vimentin, smooth muscle cell actin, and Ki-67. Authors therefore suggested that this scaffold type promoted regeneration, angiogenesis, cell proliferation and fibro-connective tissue formation [53].
We also described multiple decellularization protocols for whole sheep uterus scaffold production [54]. Interestingly, the successful rat uterus decellularization protocol previously developed by us was not efficient on the sheep uterus. This suggests that decellularization protocols need to be revised and fine-tuned for each species. The same study also suggested that an efficient decellularization of the sheep uterus can be obtained by SDC (Fig. 2), a milder detergent compared to most successful protocol established by Daryabari et al. [53]. This might be of importance since a milder detergent protocol was advantageous in rodent studies for immunological reasons and reduced the formation of DAMPs [39, 40]. We also addressed an important hurdle that is rarely discussed in bioengineering studies: to obtain an efficient recellularization of the scaffold. Most cells only remained in the superficial scaffold compartments, while a vast volume of the sheep uterus scaffold remained cell-free [54]. For these reasons, these scaffolds were further assessed in a follow-up study. Using two separate functional bioactivity assays, we were able to show that the decellularized sheep uterus tissue stimulated growth signaling pathways in neurons and promoted angiogenesis in a fertilized chicken egg assay [55]. Furthermore, pre-conditioning the scaffolds and decreasing the collagen fiber thickness prior to the recellularization step with matrix metalloproteinases 2 and 9 increased the recellularization efficiency by 200%–300%, independent of the three decellularization protocols used for the scaffold production.
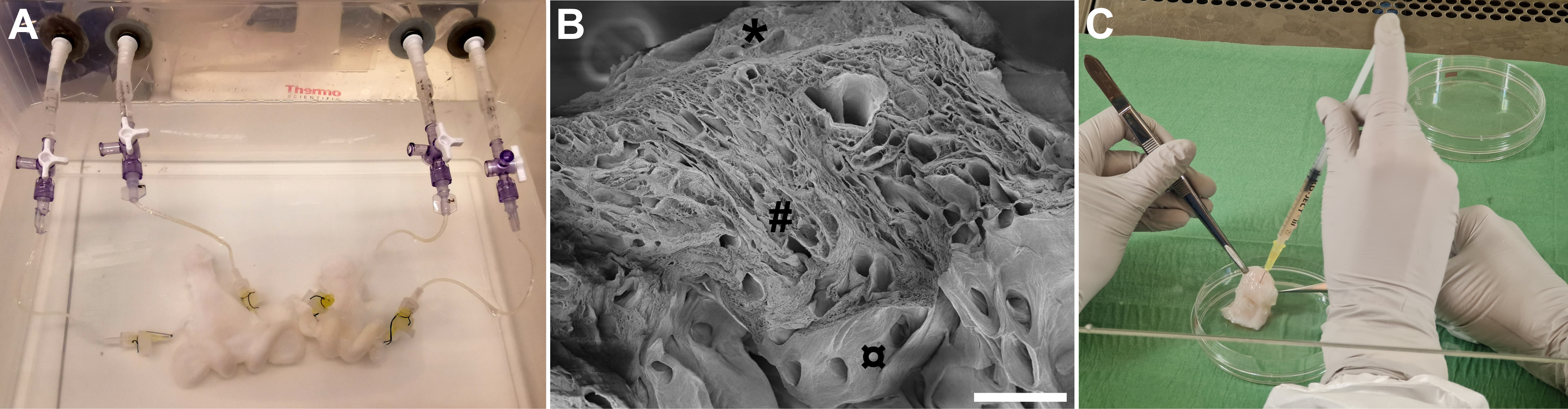
Decellularization and recellularization of sheep uterus tissue.
(A) Two sheep uteri being perfused through their uterine arteries with sodium
deoxycholate during the decellularization process. The organs are also processed
with deionized water, DNase and peracetic acid (for sterilization) for a total of
about seven days before the tissue has become decellularized. (B) Scanning
electron microscopy reveals that the remaining uterus scaffold consists of
extracellular matrix, and studies have shown that conduits for the vasculature
remain patent after decellularization. (C) Recellularization of the uterus
scaffold may be conducted through these conduits, or more commonly, cells can be
injected into the scaffolding structure as shown here. Scale bar, 200
The studies mentioned above collectively show that an organ with a similar size to the human uterus can effectively be decellularized. However, thus far, only a few studies describe human uterine bioengineering strategies.
Segments of the human myometrium was first decellularized using an ethanol,
trypsin and water protocol, that also worked well to decellularize rat myometrial
tissue [56]. These scaffolds were recellularized with a rat or a human myocyte
cell line and cultured for 51 days in vitro. Surprisingly, the human
cells integrated better in the rat scaffolds where they formed a multilayered
laminar structure. They were also able to detect contractility function of the
tissue using organ bath experiments. A more recent study decellularized human
endometrial tissue segments of 1–2 cm
Species | Scaffold | Decellularization detergent | Recellularization | In vivo | Pregnancy | Reference |
Mouse | DC uterus | SDS | No cells | 7 weeks | Yes | [36] |
Rat | Myofibroblast tissue | N/A | Myofibroblasts | 12 weeks | Yes | [28] |
Rat | Collagen | N/A | MSCs | 90 days | Yes | [29] |
Rat | Collagen | N/A | No cells | 90 days | Yes | [30] |
Rat | DC uterus | Triton X-100, DMSO or SDC | No cells | Not tested | Not tested | [31] |
Rat | DC uterus | SDS, Triton X-100/high hydrostatic pressure | No cells | 51 days | Yes | [32] |
Rat | DC uterus | SDS | Rat neonatal, endometrial cells, MSCs | 90 days | Yes | [33] |
Rat | DC uterus | TritonX-100, DMSO, SDC | GFP labelled MSCs | 9 weeks | Yes | [34] |
Rat | DC uterus | SDS | No cells | 11 weeks | Yes | [35] |
Rat | DC uterus | SDS | GFP labelled MSCs | 90 days | Yes | [37] |
Rat | DC uterus | TritonX-100, DMSO or SDC | No cells | 3 months | Not tested | [39, 40] |
Rabbit | DC uterus derived hydrogel | SDS, Triton X-100, DNase | In vitro embryo culture | Not tested | Not tested | [41] |
Rabbit | DC uterus | SDS, Triton X-100 | No cells | 90 days, xenograft (rat) | Not tested | [42] |
Rabbit | Polymer scaffold | N/A | Uterus cells | 6 months | Yes | [43] |
Pig | DC uterus | SDS, Triton X-100 | Stromal, epithelial cells | Not tested | Not tested | [44] |
Pig | DC uterus derived hydrogel | SDS, Triton X-100, DNase | human endometrial organoids | Not tested | Not tested | [45] |
Pig | DC uterus derived hydrogel | SDS, Triton X-100, DNase | No cells | 24 days, xenograft (mouse) | Yes | [46] |
Goat | DC uterus | SDC, Triton X-100 | No cells | Not tested | Not tested | [47] |
Sheep | DC uterus | SDS, Triton X-100, Formalin | No cells | 10 days, xenograft (rat) | Not tested | [53] |
Sheep | DC uterus | SDS, SDC, Triton X-100, DNase | Sheep fetal MSCs | Not tested | Not tested | [54, 55] |
Human | DC myometrium | Ethanol, trypsin | Myocytes | Not tested | Not tested | [56] |
Human | DC endometrium | SDC, Triton X-100 | Endometrial cells | Not tested | Not tested | [57] |
DC, decellularization; MSCs, mesenchymal stem cells; SDS, sodium dodecyl sulfate; SDC, sodium deoxycholate; DMSO, dimethyl sulfoxide. |
This review clearly summarizes several promising scaffold types for uterus bioengineering applications. However, the reconstruction/recellularization phase is still a challenge. This process must be improved in order to succeed with larger scaffolding types in vivo, at least when decellularized tissue is used as a scaffold. Improvements in preconditioning decellularized tissue prior to recellularization can increase scaffold stiffness and prolong its degradation time [42], assist cellular migration and subsequent graft quality [55].
Sophisticated in vitro perfusion bioreactors have been used to support extreme premature lamb [58] or support the maturation of post-implantation mouse embryos until the hindlimb formation stage up to embryonic day 11 [59]. Additionally, novel normothermic organ perfusion systems using blood-based perfusion medium have proved better than conventional organ preservation methods for liver and kidney transplantation [60, 61]. With modifications, these and other perfusion systems specifically developed for ex vivo pig, sheep and human uterus perfusion [62, 63, 64, 65], will likely prove to be of significant value for future whole uterus recellularization protocols. During this extended in vitro recellularization time, multiple cell types will need to be applied in sequence, including endothelial cells for the vasculature reconstruction [66, 67].
Additional challenges will also include to develop standardized good laboratory practice (GLP) and standard operating procedures (SOP) suitable for the development of clinical grade human uterus grafts that can be used as advanced therapeutic medical products.
Significant progress was made in uterus bioengineering during the last decade with convincing evidence from multiple independent groups. Beneficial constructs were created for partial uterine repair in rodent and rabbit models, and uterus scaffolds were developed for large animal models, in particular for the sheep model. Hence, uterus bioengineering principles should be further evaluated in relevant pre-clinical animal models, using larger and more clinically relevant grafts. Partial uterine reconstruction by bioengineered tissue transplantation is a much closer clinical reality compared with whole uterus bioengineering principles that are necessary to replace a donor in a UTx setting.
AP and MH did the literature research and drafted the early version of the manuscript, MB and MH finalized it to its current state. All authors revised and approved the final version of the manuscript.
Not applicable.
We thank the reviewers for their time and valuable comments.
The authors receive financial support to conduct uterus bioengineering research by the Knut and Alice Wallenberg foundation, the Swedish research council (VR: 116008), the ALF-agreement (between the Swedish government and the county council), the Adlerbertska, Hjalmar Svensson, and Wilhelm & Martina Lundgrens research foundations.
The authors declare no conflict of interest. MB is serving as one of the Guest editors of this journal. We declare that MB had no involvement in the peer review of this article and has no access to information regarding its peer review. Full responsibility for the editorial process for this article was delegated to JO.